The epigenetic DNA modification 5-carboxylcytosine promotes high levels of cyclobutane pyrimidine dimer formation upon UVB irradiation
Original Research Paper
Sang-In Kim & Gerd P. Pfeifer Genome Instability & Disease , 259–69(2021)
Abstract
In mammals, DNA methyltransferases create 5-methylcytosines (5mC) predominantly at CpG dinucleotides. 5mC oxidases convert 5mC in three consecutive oxidation steps to 5-hydroxymethylcytosine (5hmC), 5-formylcytosine (5fC) and then 5-carboxylcytosine (5caC). Upon irradiation with UV light, dipyrimidines containing C, 5mC and 5hmC are known to form cyclobutane pyrimidine dimers (CPDs) as major DNA photolesions. However, the photobiology of 5fC and 5caC has remained largely unexplored. Here, we tested a series of oligonucleotides with single or multiple positions carrying cytosine (C), 5mC, 5hmC, 5fC, or 5caC and irradiated them with different sources of UV irradiation. Although UVC radiation produced CPDs near dipyrimidines containing all types of modified cytosine bases, UVB radiation produced by far the highest levels of CPDs near 5caC-containing sequences. Dipyrimidines one or two nucleotide positions adjacent to 5caC but not always those involving this modified base directly were the major sites for these prominent UVB photoproducts. This selectivity did not depend on whether 5caC was present on one or both DNA strands at CpG sequences. We also observed a tendency of the 5caC-containing DNA strands to undergo apparent covalent crosslinking. This reaction occurred with UVB or UVC, but not with UVA irradiation. Our data show that 5-carboxylcytosine, although generally a rare base in the genome, can nonetheless make a strong contribution to sequence-specific DNA damage perhaps by acting as a DNA-intrinsic photosensitizer.
Introduction
Ultraviolet light from the sun has been a threat to genome stability ever since the emergence of DNA-containing organisms. The solar UV spectrum is conventionally divided into different spectral components, UVC (100–280 nm), UVB (280–315 nm) and UVA (315–400 nm). The shorter wavelengths below approximately 300 nm are absorbed effectively by the atmosphere and do not reach the surface of the earth. However, the UVB component between 300 and 315 nm can penetrate the atmosphere and can cause DNA damage in exposed cells. It is this type of UVB irradiation that has been prominently linked to skin cancer and other adverse health effects in humans (Pfeifer 2020).
Direct damage to DNA by ultraviolet radiation leads to the formation of dimeric pyrimidine photoproducts such as thymine dimers as reported about 60 years ago (Beukers and Berends 1960; Johns et al. 1962; Setlow and Setlow 1962; Wacker et al. 1962). UVB radiation causes the dimerization of two adjacent pyrimidine bases as the most abundant DNA photoproduct (Pfeifer 2020). The UV dimer formation is initiated by absorption of photons by the DNA bases and occurs through the formation of electronic excited states (Markovitsi 2016). The most prominent UVB-induced dimers in double-stranded DNA are the cis-syn cyclobutane pyrimidine dimers (CPDs), followed by the less frequent pyrimidine (6–4) pyrimidone photoproducts (Mitchell and Fernandez 2011; Cadet et al. 2012; Pfeifer 2020). The dimerization occurs via the C5, C6 double bonds of the two adjacent pyrimidine bases. The CPDs also form very efficiently when UVC irradiation at 254 nm, e.g., from germicidal lamps, is used. However, this radiation is not relevant for exposures on the earth’s surface. On the other hand, longer wavelength UVA light sources can also generate CPDs, albeit at frequencies that are orders of magnitude smaller than those achieved with UVB radiation (Douki et al. 2003; Rochette et al. 2003; Besaratinia et al. 2005). Using solar UV simulators as equipment to mimic radiation from the sun on the earth’s surface, CPDs are clearly the major DNA damage product observed in irradiated DNA or cells (Sage et al. 1996; Yoon et al. 2000).
In addition to the four standard bases, mammalian and other genomes contain a few intrinsically modified DNA bases. These bases are not the products of DNA damage but are created enzymatically by post-replication modification processes. For many decades, 5-methylcytosine (5mC) was thought to be the only such modified DNA base in mammals. Three active DNA methyltransferase enzymes (DNMT1, DNMT3A, and DNMT3B) produce 5mC predominantly at 5′CpG dinucleotide sequences. When the DNA base flanking a methylated CpG is a pyrimidine in the 5′ direction, the sequence will contain dipyrimidines with 5mC (5′TmCG or 5′CmCG at the trinucleotide level). We and others discovered earlier that these 5mC-containing dipyrimidines are particularly susceptible to form CPDs in the UVB (but not UVC) wavelength range (Drouin and Therrien 1997; Tommasi et al. 1997; Rochette et al. 2009). Interestingly, as shown by mutation reporter assays, these methylated trinucleotides tend to form mutational hotspots in UVB-irradiated cells (You et al. 1999, 2000; You and Pfeifer 2001), a phenomenon also observed in the TP53 tumor suppressor gene, which contains a number of such methylated UVB-sensitive trinucleotides (Tornaletti and Pfeifer 1995; Tommasi et al. 1997). The increased tendency of 5mC to form CPDs has been ascribed to the redshift in UV absorption of the methylated cytosine as compared to cytosine (You et al. 1999). Other mechanisms may also contribute. 5mC has a tenfold higher fluorescence lifetime than C, and the methyl group could affect DNA duplex conformation to make dimerization more likely (Sharonov et al. 2003; Banyasz et al. 2016; Martinez-Fernandez et al. 2017).
About a decade ago, an additional modified base, 5-hydroxymethylcytosine (5hmC), was discovered in mammalian DNA as a product of an enzymatic oxidation reaction that is catalyzed by the ten-eleven-translocation (TET) protein family (Tahiliani et al. 2009). These enzymes oxidize 5mC to 5hmC and then proceed with additional oxidation steps to produce 5-formylcytosine (5fC) and then 5-carboxylcytosine (5caC) as the final product (He et al. 2011; Ito et al. 2011). The two bases 5fC and 5caC are recognized by the base excision repair enzyme TDG (thymine DNA glycosylase), which excises them, leaving an abasic site (He et al. 2011). The completion of the repair pathway by base excision repair leads to the restoration of cytosine at initially methylated CpG sites. 5hmC is moderately abundant in several cell types (such as neurons or embryonic stem cells) where it seems to act as a cell identity mark often accumulating at enhancer regions and in gene bodies (Colquitt et al. 2013). This modified base is found in skin-derived cells including keratinocytes, fibroblasts, and melanocytes (Kim et al. 2013). We showed earlier that different sources of UV, including UVC and UVB radiation can produce CPDs containing 5hmC. However, formation of CPDs at dipyrimidines containing 5hmC was not enhanced relative to cytosine or 5mC at the same sequence positions (Kim et al. 2013).
The photobiological properties of 5fC and 5caC are largely unknown. Even though the levels of 5fC and 5caC are much lower than those of 5mC and 5hmC, we hypothesized that these DNA bases may undergo photochemical reactions and CPD formation in a manner perhaps different from the other cytosine-derived bases. To test their susceptibility to CPD formation, we incorporated these modified cytosines into oligonucleotides in different DNA sequence contexts, but always including their natural context, which involves a 5′CpG. We show that the carbonyl-containing cytosine derivatives, in particular 5caC, promote efficient CPD formation in a targeted and in a semi-targeted manner, and these positions may represent major sites of UVB-induced DNA damage.
Results
5caC promotes enhanced CPD formation at neighboring dipyrimidines after UVC irradiation
We initially hypothesized that the various modified forms of the DNA base cytosine may have different susceptibilities to form cyclobutane pyrimidine dimers when part of dipyrimidine sequences. In order to study CPD formation at these bases in vitro, we created a series of oligonucleotides that contain either C, 5mC, 5hmC, 5fC, or 5caC (Fig. 1) at CpG sequences and another pyrimidine at the 5′ position to form 5′TXG or 5′CXG sequences, where X represents the modified or unmodified cytosine. We began with 3′-biotin-labeled 64-mer oligonucleotides that contained 5′TCXG and 5′TTTTXG. This oligonucleotide also contained a sequence 5′TTAXGXG (Fig. 2a). These oligonucleotides were first irradiated with different doses of UVC irradiation (Fig. 2). In a control experiment, the oligonucleotides were subjected to denaturing polyacrylamide electrophoresis in the absence of enzymatic processing (Fig. 2b). This experiment showed a small number of cleaved fragments at the positions of the modified bases but only for the samples that contained 5fC or 5caC, and this was independent of whether the samples were irradiated or not (Fig. 2b). This degradation is likely due to the relative instability of DNA bases containing 5fC or 5caC versus the other base modifications.
Fig. 1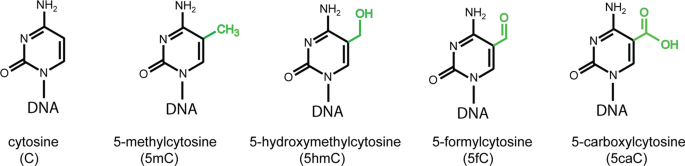
Structures of the C, 5mC, 5hmC, 5fC, and 5caC bases
Full size imageFig. 2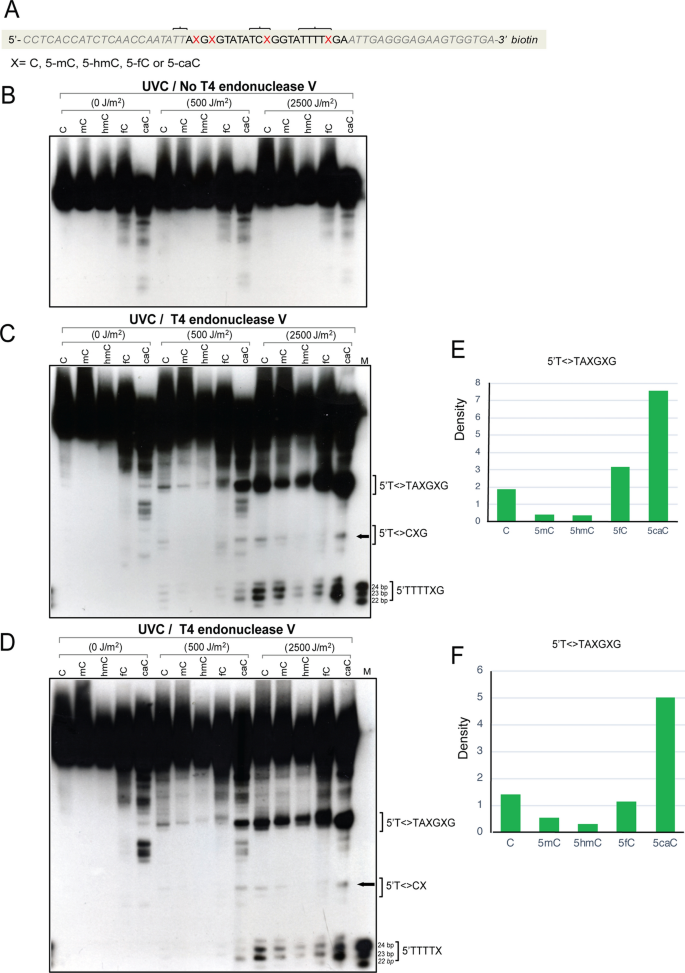
Formation of UVC-induced CPDs in 64-mer oligonucleotides containing cytosine, 5-methylcytosine, 5-hydroxymethylcytosine, 5-formylcytosine, or 5-carboxylcytosine. a Sequence of the 64-mers containing C, 5mC, 5hmC, 5fC, or 5caC at CpG positions. X marks the position of the modified cytosine. The oligonucleotides were labeled with biotin at the 3′ end. The brackets indicate sites of CPD formation as shown in panels c and d. b The oligonucleotides were irradiated with different doses of UVC and then separated on a 10% denaturing polyacrylamide gel. This gel shows the control samples without T4 endonuclease V cleavage. c The oligonucleotides were irradiated with different doses of UVC, cleaved with T4 endonuclease V and then separated on a 10% denaturing polyacrylamide gel. The positions of CPDs near the modified cytosine bases are indicated by brackets. The positions marked T<>T or T<>C on the right side of the gel indicates CPD formation, for example at the 5′TT sequence of 5′TTAXGXG. In this experiment, both DNA strands carried the modified bases. d The modified base containing sense-strand 64-mers were annealed with unmodified antisense strand to form hemi-modified double-stranded DNA. The oligonucleotides were irradiated with different doses of UVC, cleaved with T4 endonuclease V and then separated on a 10% denaturing polyacrylamide gel. The positions of CPDs containing the modified cytosine bases are indicated. e and f Quantitation of CPDs at the 5′T<>TAXGXG sequences. The images were quantitated, and background was subtracted. e Fully modified DNA (panel c). f Hemi-modified DNA (panel d)
Full size imageWe used doses of UV radiation that can effectively form CPDs as shown previously by immunodot blots with anti-CPD antibodies (Kim et al. 2013). Next, we digested the oligonucleotides by incubation with an excess amount of T4 endonuclease V after irradiation, a DNA glycosylase which cleaves CPDs specifically and sequence-independently and also has AP lyase activity to produce incision of the resulting abasic site (Yasuda and Sekiguchi 1970; Dodson and Lloyd 1989). In denaturing gels, the strand breaks indicate CPD sites. We observed strong levels of CPD formation at the 5′TT of the 5′TTAXGXG sequence and also at 5′TTTTXG. When compared with CPDs containing cytosine, there was a lower level of CPDs at these positions when the bases were 5mC, 5hmC, or 5fC (Fig. 2c). However, the highest levels of CPDs were clearly formed near sequences that contain 5caC, which was found at all three modified C positions analyzed. Unexpectedly, the high damage signal in these 5caC oligonucleotides did not occur at the dipyrimidine position closest to the guanine (i.e., at 5′T<>XG or 5′C<>XG). Instead, it occurred one dipyrimidine position further away towards the 5′ direction (for example at 5′T<>TXG or 5′T<>CXG). Also, unexpectedly, the sequence 5′TTAXGXG, which does not even contain a dipyrimidine with the base X (5caC) shows very high levels of CPDs near 5′T<>T, with the 5caC base not being directly involved in dimerization. Panel c shows the data with both DNA strands containing the modified bases. In panel d, an unmodified strand was annealed to the modified base-containing strand. The results with fully modified and hemi-modified DNA were similar. These data indicate that 5caC can direct semi-targeted CPD formation in its immediate vicinity but not involving the 5caC base itself.
The relative susceptibility of 5caC-containing sequences to form CPDs is even more pronounced with UVB
In the next set of experiments, we used UVB irradiation at a peak wavelength of 312 nm, instead of UVC. As shown in Fig. 3, panels c, d, e, and f, the CPD frequency near 5caC sites is about tenfold or more increased as compared to the same oligonucleotides containing cytosine, 5mC, or 5hmC, and is also about twice as high as with 5fC. Again, this effect did not depend on whether the modified bases were present on both DNA strand or on one DNA strand only. As with UVC, the CPD signal was dis-localized by one base position (or two base positions at the 5′TTAXG sequence) away from the 5caC base.
Fig. 3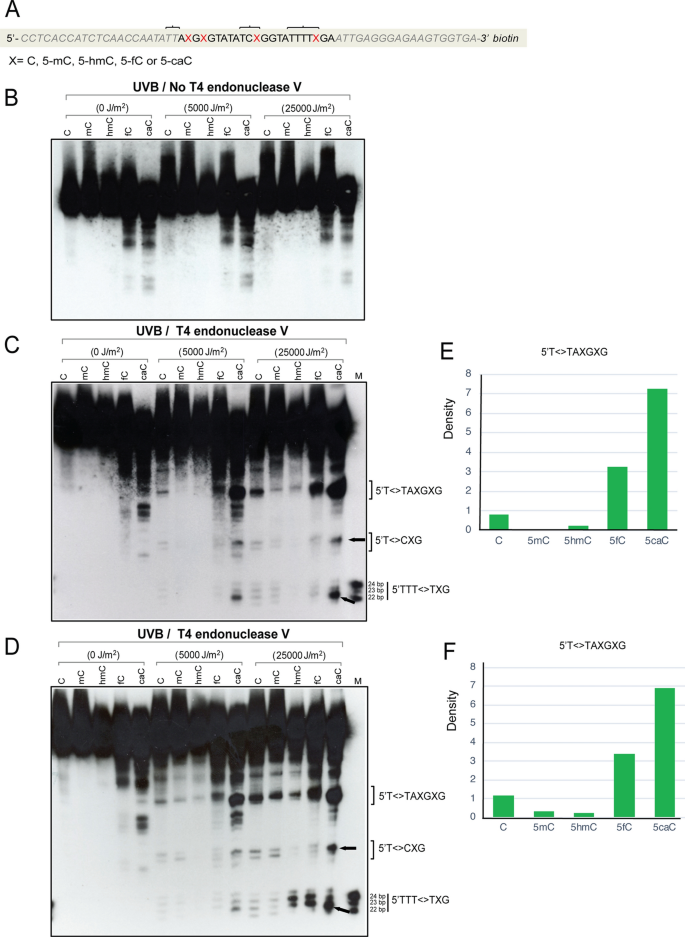
Formation of UVB-induced CPDs in 64-mer oligonucleotides containing cytosine, 5-methylcytosine, 5-hydroxymethylcytosine, 5-formylcytosine, or 5-carboxylcytosine. a Sequence of the 64-mers containing C, 5mC, 5hmC, 5fC, or 5caC at positions X. The oligonucleotides were labeled with biotin at the 3′ end. The brackets indicate sites of CPD formation as shown in panels c and d. b The oligonucleotides were irradiated with different doses of UVB and then separated on a 10% denaturing polyacrylamide gel without T4 endonuclease V cleavage. c The oligonucleotides were irradiated with different doses of UVB, cleaved with T4 endonuclease V and then separated on a 10% denaturing polyacrylamide gel. The positions of CPDs near the modified cytosine base are indicated by brackets. The positions marked T<>T or T<>C on the right side of the gel indicates CPD formation, for example at the 5′TT sequence of 5′TTAXGXG. In this experiment, both DNA strands carried the modified bases. d The modified base containing sense strand 64-mers were annealed with unmodified antisense strand to form hemi-modified double-stranded DNA. The oligonucleotides were irradiated with different doses of UVB, cleaved with T4 endonuclease V and then separated on a 10% denaturing polyacrylamide gel. The positions of CPDs containing the modified cytosine base are indicated by brackets. e and f Quantitation of CPDs at the 5′T<>TAXGXG sequences. The images were quantitated, and background was subtracted. e Fully modified DNA (panel c). f Hemi-modified DNA (panel d)
Full size imageA different labeling strategy shows similar results
Because the results with the 5caC oligonucleotide were unexpected, we shifted to a different labeling strategy where the 5′ ends of the oligonucleotides were labeled with 32P and T4 polynucleotide kinase (Fig. 4a). Also, in this case, 5caC pinpointed the sites of highest levels of CPD formation. This difference was clearly most pronounced with UVB irradiation (Fig. 4b, left panel) as compared to UVC irradiation (Fig. 4b, right panel). Because this experiment used 5′ labeling and 32P, the resolution near the top of the gel was not sufficient to indicate which exact dipyrimidine was involved, but the overall pattern is similar to the biotin labeling study showing enhanced CPD formation near 5caC sites. We also included a high dose of UVA irradiation in this set of experiments (Fig. 4c). However, aside from the non-UV-specific degradation bands of the 5fC- and 5caC-containing oligonucleotides, we did not obtain a specific signal of CPD formation with UVA.
Fig. 4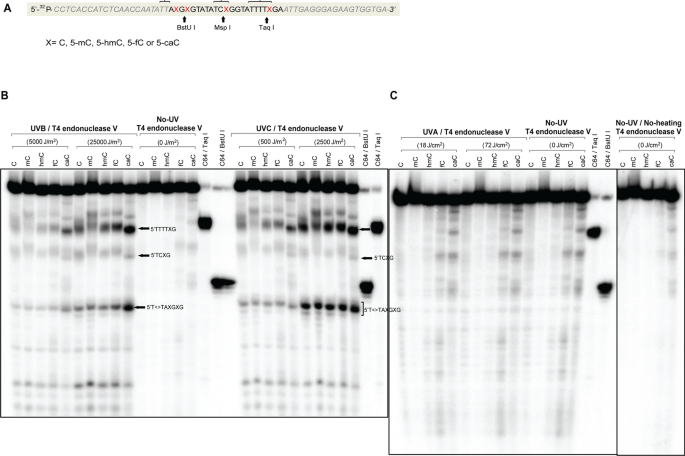
Formation of CPDs in 64-mer oligonucleotides containing cytosine, 5-methylcytosine, 5-hydroxymethylcytosine, 5-formylcytosine or 5-carboxylcytosine. a Sequence of the 64-mers containing C, 5mC, 5hmC, 5fC, or 5caC at positions X. The oligonucleotides were labeled with [γ-32P]ATP at the 5′ end. Positions of restriction endonuclease cleavage sites are indicated. The brackets indicate sites of CPD formation as shown in panel b. b The oligonucleotides were irradiated with different doses of UVB or UVC, cleaved with T4 endonuclease V and then separated on a 10% denaturing polyacrylamide gel. The positions of CPDs containing the modified cytosine base are indicated by arrows. c The oligonucleotides were irradiated with different doses of UVA, cleaved with T4 endonuclease V and then separated on a 10% denaturing polyacrylamide gel
Full size imageSingle dipyrimidine sequences with 5caC can form CPDs
We next used 45-mer oligonucleotides that contain only a single modified cytosine at a CpG sequence (Fig. 5a). This sequence showed low background cleavage in the absence of UV irradiation and in the absence of T4 endonuclease V cleavage (Fig. 5b). When irradiated with UVB, the 5′TXG sequence in the middle of the oligonucleotide showed the strongest T4 endonuclease cleavage signal when the base X was a 5mC or a 5caC (Fig. 5c). Because there is only one dipyrimidine near this position (5′TX) (Fig. 5a), the data suggest that 5′TcaC sequences are capable of direct and efficient CPD formation. We also used an oligonucleotide with a central 5′CXG sequence with UVB irradiation. In this case, only very weak signals were obtained, and these were most pronounced at 5′CmC sequences, followed by 5′CC, but were almost undetectable at 5′ChmC, 5′CfC, and 5′CcaC (Figure S1).
Fig. 5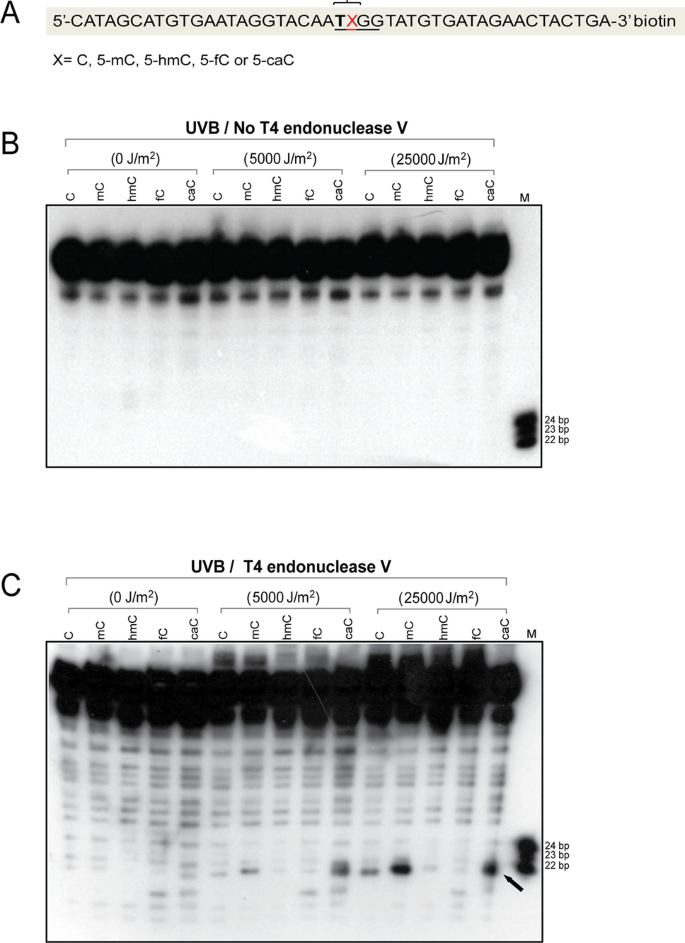
Formation of CPDs in 45-mer oligonucleotides containing cytosine, 5-methylcytosine, 5-hydroxymethylcytosine, 5-formylcytosine, or 5-carboxylcytosine. a Sequence of the 45-mers containing C, 5mC, 5hmC, 5fC, or 5caC at positions X. The oligonucleotides were labeled with biotin at the 3′ end. The bracket indicates sites of CPD formation as shown in panel c. b The oligonucleotides were irradiated with different doses of UVB and then separated on a 10% denaturing polyacrylamide gel without T4 endonuclease V cleavage (control). c The oligonucleotides were irradiated with different doses of UVB, cleaved with T4 endonuclease V and then separated on a 10% denaturing polyacrylamide gel. The position of CPDs containing the modified cytosine base is indicated by an arrow. Both DNA strands of the duplex DNA carried the modified DNA base. The experiment was repeated three times with similar results
Full size imageUVB induces higher molecular weight forms (potential crosslinks) of oligonucleotides containing 5-carboxylcytosine
When gels with UVB-irradiated 5caC-containing oligonucleotides were run for longer times, we noticed distinct higher molecular weight forms suggesting a crosslinking event that is resistant to heating and the denaturing gel electrophoresis conditions (Fig. 6). The shifted bands appeared with both fully modified and hemi-modified 5caC sequences (Fig. 6). These bands were visible with UVB and UVC irradiation and were seen also in the absence of T4 endonuclease V cleavage (Fig. S2). Although we did not determine the sequence composition or structure of these higher molecular weight species, we noticed that they formed prominently only when 5caC was present in the oligonucleotides. The phenomenon was most pronounced with the 64-mer sequences, which contain four 5caC bases on each DNA strand as opposed to the 45-mer, which contain only one 5caC and formed lower amounts of the higher molecular weight species (Figs. S3, S4). The shifted bands became visible with either biotin labeling (Figs. 6, S2, S3) or with 32P labeling (Fig. S4). The mobility of these bands suggested a dimerization reaction, perhaps due to an unusual photochemical reaction of 5caC. The product was seen when both DNA strands carried the 5caC bases or when only one strand (the labeled strand) contained this modified base (Fig. 6). The high molecular weight species may represent interstrand crosslinks or crosslinks between two separate duplex DNA molecules.
Fig. 6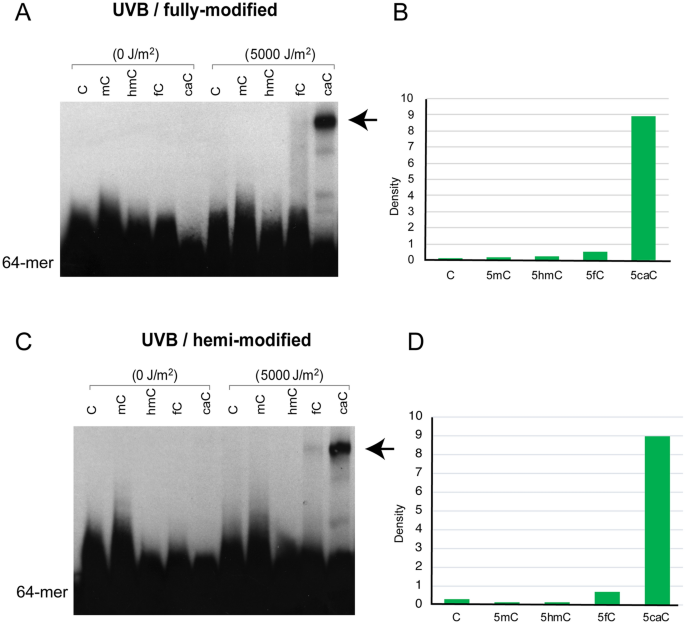
A putative crosslink of 5caC-containing DNA sequences after UVB irradiation occurs in fully modified and hemi-modified DNA molecules. a UVB irradiation was used to induce crosslinked molecules from 5caC sequences. Both DNA strands carried the modified bases. b Quantitation of the putative crosslink band in the oligonucleotides with different modified bases. c UVB irradiation was used to induce crosslinked molecules from 5caC sequences. Only one DNA strand carried the modified bases. d Quantitation of the putative crosslink band in the oligonucleotides with different modified bases. The position of the putative crosslink is indicated by arrows
Full size imageDiscussion
The DNA damage forming properties of UV radiation has been investigated extensively in previous studies. The most frequent DNA lesion induced by UVC and UVB radiation is the CPD. (6–4)PPs also form with UVC and shorter wavelength UVB radiation (280–300 nm) (Pfeifer 2020). UVA, although capable of inducing CPDs at very high irradiation doses, may also produce oxidative DNA damage, for example in the form of 8-oxo-guanine (Cadet and Douki 2011; Cadet et al. 2012, 2015). In mutagenesis experiments in which either CPDs or (6–4)PPs were removed before performing the mutation assays, it has been determined that the CPD is the more mutagenic lesion (You et al. 2001). CPDs form preferentially at dipyrimidines containing thymine as the 5′ base, such as 5′TT and 5′TC and form at lower levels at sequences that have cytosine as the 5′ base (5′CT and 5′CC) (Pfeifer 2020). We showed earlier that the 5mC bases forming at CpG dinucleotides are prone to enhanced CPD formation when part of a dipyrimidine (Tommasi et al. 1997), and these sequences are also mutation hotspots in experimental in vivo systems (You and Pfeifer 2001). The latter phenomenon is likely related to enhanced CPD formation in the UVB range and effective deamination of 5mC to T when the base is part of a CPD (Lee and Pfeifer 2003; Vu et al. 2006; Cannistraro et al. 2015).
The ability of 5hmC to form CPDs has been shown previously when it was determined that some sequences with 5hmC are less prone to dimer formation than when 5mC or cytosine bases are present (Kim et al. 2013). This property of 5hmC was confirmed in the present study. The ability of 5fC and 5caC to form UV-induced CPD sites has remained unclear. We show that 5fC behaves similar to 5hmC in its ability to form lower levels of CPDs involving the 5fC base (Fig. 5). On the other hand, DNA containing 5caC had the most unusual photo-reactivity. First, we observed that 5caC itself can be part of a CPD and these form at levels comparable to those containing 5mC (Fig. 5c). Second, the presence of 5caC promoted the formation of CPDs one to two nucleotide positions away from the 5caC base itself, for example at the TT sequence within the context 5′TTAcaCG (Figs. 2, 3, 4). This phenomenon was most pronounced with UVB irradiation. Third, oligonucleotides containing 5caC, but not the other modified cytosine derivatives, formed higher molecular weight crosslinked species.
The photochemical basis for this unusual photo-reactivity of 5caC remains unknown. Because of a prominent red shift of 5fC (13 nm) and 5caC (10 nm) relative to cytosine, these two modified bases are expected to be excited much more easily than the canonical DNA bases when exposed to UVB or solar radiation (Wang et al. 2019). However, this difference alone does not explain the unique susceptibility of sequences with 5caC relative to 5fC to form CPDs near the modified base. Through intersystem crossing involving a triplet state, 5fC can induce substantial amounts of singlet oxygen and its associated oxidative DNA damage (Wang et al. 2019), which we did not examine in the present study. Wang et al. also determined that 5caC can dissipate excited-state energy on an ultrafast time scale, suggesting that the base should be stable under UV irradiation. It has emerged in recent years that the formation of excited states in DNA by UV radiation is an essential pathway leading to photochemical base dimerization (Banyasz et al. 2012). Excited states can be delocalized over two or more stacked DNA bases and can be of either Frenkel exciton (ππ*) or of a charge transfer type or a mix of both (Takaya et al. 2008; Schreier et al. 2015; Markovitsi 2016). The degree of delocalization of excited states as well as the mechanisms of how excited states undergo relaxation have remained under debate. High-energy emitting long-lived mixed (HELM) states can extend across both DNA strands in a duplex molecule (Huix-Rotllant et al. 2015).
What we observed here is strongly enhanced formation of CPDs that was delocalized by 1–2 bases away from the 5caC. We do not currently understand the photochemical mechanism of how 5caC may modify the formation and dynamics of excited electronic states. One other important parameter that influences successful CPD formation is a DNA structure that favors proximity of the C5–C6 double bonds of two adjacent pyrimidines. However, the current data suggest that 5caC has no major effect on DNA structure (Ngo et al. 2016) although base pairing may be weakened within 5fC and 5caC base pairs (Dai et al. 2016). We speculate that the carboxyl group of 5caC may easily form singlet or triplet state carbonyls with a delocalized excited state where the energy could be transferred to nearby bases, perhaps analogous to the action of photosensitizers, such as acetone or acetophenone (Premi and Brash 2016). In this sense, 5caC could be viewed as a DNA-intrinsic photosensitizer, in a manner conceptually similar to the DNA damage products 5-formyluracil (Aparici-Espert et al. 2018) or the (6–4) photoproduct (Vendrell-Criado et al. 2016), which both enhance the formation of CPDs via the triplet excited state. However, the exact mechanism underlying our observations with 5caC and also the pathways leading to apparent crosslink formation of the 5caC-containing DNAs remain to be established by biophysical studies.
5caC is generally thought of as an intermediate reaction product in TET protein-induced DNA demethylation reactions along the DNA base sequence 5mC → 5hmC → 5fC → 5caC → C. When measured using sensitive mass-spectrometry-based approaches, its levels are very low in most tissues analyzed (Globisch et al. 2010). Differentiating cells that undergo a transition from a stem or progenitor cell are characterized by TET-induced DNA demethylation at many tissue-specific enhancer regions (Hahn et al. 2019), and in this situation, 5caC would be expected to be present at much higher levels. In fact, an accumulation of 5caC at enhancers has been observed in embryonic stem cells (Shen et al. 2013). Therefore, we propose that the UVB-induced selective targeting of enhancer sequences carrying 5caC may be particularly relevant in skin cells that are in a state of early differentiation, for example during the transition of basal cells in the epidermis to mature keratinocytes, or during the transition of melanocyte precursors to fully differentiated melanocytes. This cell population represents a critical fraction of cells in the skin that may be most susceptible to transformation, and mutations related to 5caC-selective DNA damage at enhancers could therefore have biological significance. Further studies of the photochemical and photobiological properties of this unique DNA base are justified to examine these putative mechanisms.
Materials and methods
Oligonucleotides
All site-specifically modified oligonucleotides were synthesized at the W.M. Keck oligonucleotide synthesis facility at Yale University. The modified oligonucleotides (64-mer: 5′- CCTCACCATCTCAACCAATATTACGCGTATATCCGGTATTTTCGAATT-GAGGGAGAAGTGGTGA or 45-mers: 5′-CATAGCATGTGAATAGGT-ACAATCGGTATGTGATAGAACTACTGA and 5′-CATAGCATGTGAATAGG-TACAACCGGTATGTGATAGAACTACTGA) contained C, 5mC, 5hmC, 5fC, or 5caC at the underlined 5′-CpG dinucleotide positions. Complementary strand oligonucleotides also containing the modified bases at the equivalent 5′-CpG sites were used for annealing to form double-stranded DNA. To test oligonucleotides that were modified on only one strand, we annealed unmodified, cytosine-containing oligonucleotides to the modified strand.
Preparation of end-labeled oligonucleotides
The 3′-biotin labeling of oligonucleotides was performed with terminal deoxynucleotidyl transferase (Invitrogen; Carlsbad, CA) and biotin-16-ddUTP (Roche; Indianapolis, IN) using the following conditions. Three hundred nanograms of each sense strand oligonucleotide was incubated at 37 °C for 30 min in 50 µl reaction mixture containing 15 units of terminal deoxynucleotidyl transferase, 2 µM biotin-16-ddUTP, 0.1 M potassium cacodylate (pH 7.2), 2 mM CoCl2, and 0.2 mM dithiothreitol. After this incubation, 1 µl of 0.5 M EDTA (pH 8.0) and 50 µl of chloroform:isoamyl alcohol (24:1) were added to the reaction mixture. The mixture was centrifuged at 13,000×g for 3 min, and then the upper phase was recovered and precipitated with ethanol. The 3′-biotin labeled sense-strand oligonucleotides were annealed to unlabeled antisense-strand oligonucleotides. For the 5′-32P labeling, one microgram of each oligonucleotide was phosphorylated at 37 °C for 1 h with T4 polynucleotide kinase (New England Biolabs; Ipswich, MA) in the presence of [γ-32P]ATP and purified on G-50 spin columns (Roche; Indianapolis, IN). The 5′-32P-labeled sense-strand oligonucleotide was annealed to an unlabeled oligonucleotide representing the opposite strand.
UV irradiation
The end-labeled double-stranded oligonucleotides were irradiated in water on parafilm placed directly on ice to avoid heat production. A Sellas Sunlight System (Medizinische Geräte GmbH; Gevelsberg, Germany) was used for UVA irradiation at doses of 18 J/cm2 and 72 J/cm2. This UVA source exclusively emits long-wave UVA (UVA1: 340–400 nm) with an average fluence rate of 60 mW/cm2. The UVB irradiation was carried out with three fluorescent tubes (Philips TL 20W/12R, peak spectral emission at 312 nm). The UVB doses were 5000 and 25,000 J/m2 as determined with a UVX radiometer (Ultraviolet Products; Upland, CA). The UVC irradiation at doses of 500 and 2500 J/m2 was performed using five 254 nm UV bulbs from a distance of 20 cm (Stratalinker UV Crosslinker 2400, Stratagene).
Mapping of CPDs at modified cytosine-containing oligonucleotides
To cleave UV-irradiated oligonucleotides at the sites of cyclobutane pyrimidine dimers, the irradiated oligonucleotides were digested with T4 endonuclease V (Trevigen; Gaithersburg, MD) under the following conditions: The enzyme digestion was completed for 3 h at 37 °C in 20 µl of reaction buffer (25 mM sodium phosphate, pH 6.8, 1 mM EDTA, 100 mM NaCl, 1 mM dithiothreitol, 0.01% Triton X-100, 0.1 mg/ml BSA) with 20 units of T4 endonuclease V. After T4 endonuclease V cleavage, the sample was added to 94% formamide loading dye (94% formamide, 2 mM EDTA, pH 8.0, 0.05% xylene cyanole, 0.05% bromophenol blue), and then denatured at 95 °C for 2 min before separation on a 10% polyacrylamide gel containing 7 M urea. The gel was run until the xylene cyanole dye reached the bottom of the gel. For the 3′-biotin-labeled samples, the gel was blotted onto a charged nylon-based membrane (Perkin Elmer; Waltham, MA) using an electrotransfer device (Trans blot SD; Bio-Rad; Hercules, CA). The signal was detected by using the Lightshift Chemiluminescent EMSA Kit (ThermoFisher Scientific; Kalamazoo, MI) following the manufacturer’s instructions. ImageJ software was used for band quantification. For the 5′-32P-labeling samples, the gel was dried and exposed to a phosphorimager screen (Molecular Dynamics; Sunnyvale, CA) or an X-ray film (Bioland; Paramount, CA) to detect signals.
References
Aparici-Espert, I., Garcia-Lainez, G., Andreu, I., Miranda, M. A., & Lhiaubet-Vallet, V. (2018). Oxidatively generated lesions as internal photosensitizers for pyrimidine dimerization in DNA. ACS Chemical Biology, 13(3), 542–547. https://doi.org/10.1021/acschembio.7b01097.
Banyasz, A., et al. (2012). Electronic excited states responsible for dimer formation upon UV absorption directly by thymine strands: joint experimental and theoretical study. Journal of the American Chemical Society, 134(36), 14834–14845. https://doi.org/10.1021/ja304069f.
Banyasz, A., et al. (2016). Effect of C5-methylation of cytosine on the UV-induced reactivity of duplex DNA: conformational and electronic factors. The Journal of Physical Chemistry B, 120(18), 4232–4242. https://doi.org/10.1021/acs.jpcb.6b03340.
Besaratinia, A., et al. (2005). DNA lesions induced by UV A1 and B radiation in human cells: comparative analyses in the overall genome and in the p53 tumor suppressor gene. Proceedings of the National Academy of Sciences of the United States of America, 102(29), 10058–10063.
Beukers, R., & Berends, W. (1960). Isolation and identification of the irradiation product of thymine. Biochimica et Biophysica Acta, 41, 550–551. https://doi.org/10.1016/0006-3002(60)90063-9.
Cadet, J., & Douki, T. (2011). Oxidatively generated damage to DNA by UVA radiation in cells and human skin. Journal of Investigative Dermatology, 131(5), 1005–1007. https://doi.org/10.1038/jid.2011.51.
Cadet, J., Douki, T., & Ravanat, J. L. (2015). Oxidatively generated damage to cellular DNA by UVB and UVA radiation. Photochemistry and Photobiology, 91(1), 140–155. https://doi.org/10.1111/php.12368.
Cadet, J., Mouret, S., Ravanat, J. L., & Douki, T. (2012). Photoinduced damage to cellular DNA: direct and photosensitized reactions. Photochemistry and Photobiology, 88(5), 1048–1065. https://doi.org/10.1111/j.1751-1097.2012.01200.x.
Cannistraro, V. J., Pondugula, S., Song, Q., & Taylor, J. S. (2015). Rapid deamination of cyclobutane pyrimidine dimer photoproducts at TCG sites in a translationally and rotationally positioned nucleosome in vivo. Journal of Biological Chemistry, 290(44), 26597–26609. https://doi.org/10.1074/jbc.M115.673301.
Colquitt, B. M., Allen, W. E., Barnea, G., & Lomvardas, S. (2013). Alteration of genic 5-hydroxymethylcytosine patterning in olfactory neurons correlates with changes in gene expression and cell identity. Proceedings of the National Academy of Sciences of the United States of America, 110(36), 14682–14687. https://doi.org/10.1073/pnas.1302759110.
Dai, Q., Sanstead, P. J., Peng, C. S., Han, D., He, C., & Tokmakoff, A. (2016). Weakened N3 hydrogen bonding by 5-formylcytosine and 5-carboxylcytosine reduces their base-pairing stability. ACS Chemical Biology, 11(2), 470–477. https://doi.org/10.1021/acschembio.5b00762.
Dodson, M. L., & Lloyd, R. S. (1989). Structure–function studies of the T4 endonuclease V repair enzyme. Mutation Research, 218(2), 49–65. https://doi.org/10.1016/0921-8777(89)90011-6.
Douki, T., Reynaud-Angelin, A., Cadet, J., & Sage, E. (2003). Bipyrimidine photoproducts rather than oxidative lesions are the main type of DNA damage involved in the genotoxic effect of solar UVA radiation. Biochemistry, 42(30), 9221–9226. https://doi.org/10.1021/bi034593c.
Drouin, R., & Therrien, J. P. (1997). UVB-induced cyclobutane pyrimidine dimer frequency correlates with skin cancer mutational hotspots in p53. Photochemistry and Photobiology, 66(5), 719–726. https://doi.org/10.1111/j.1751-1097.1997.tb03213.x.
Globisch, D., et al. (2010). Tissue distribution of 5-hydroxymethylcytosine and search for active demethylation intermediates. PLoS ONE, 5(12), e15367. https://doi.org/10.1371/journal.pone.0015367.
Hahn, M. A., et al. (2019). Reprogramming of DNA methylation at NEUROD2-bound sequences during cortical neuron differentiation. Sciences Advances, 23, eaax0080.
He, Y. F., et al. (2011). Tet-mediated formation of 5-carboxylcytosine and its excision by TDG in mammalian DNA. Science, 333(6047), 1303–1307. https://doi.org/10.1126/science.1210944.
Huix-Rotllant, M., Brazard, J., Improta, R., Burghardt, I., & Markovitsi, D. (2015). Stabilization of mixed Frenkel-charge transfer excitons extended across both strands of guanine-cytosine DNA duplexes. The Journal of Physical Chemistry Letters, 6(12), 2247–2251. https://doi.org/10.1021/acs.jpclett.5b00813.
Ito, S., et al. (2011). Tet proteins can convert 5-methylcytosine to 5-formylcytosine and 5-carboxylcytosine. Science, 333(6047), 1300–1303. https://doi.org/10.1126/science.1210597.
Johns, H. E., Rapaport, S. A., & Delbrueck, M. (1962). Photochemistry of thymine dimers. Journal of Molecular Biology, 4, 104–114. https://doi.org/10.1016/s0022-2836(62)80042-4.
Kim, S. I., Jin, S. G., & Pfeifer, G. P. (2013). Formation of cyclobutane pyrimidine dimers at dipyrimidines containing 5-hydroxymethylcytosine. Photochemical and Photobiological Sciences, 12(8), 1409–1415. https://doi.org/10.1039/c3pp50037c.
Lee, D. H., & Pfeifer, G. P. (2003). Deamination of 5-methylcytosines within cyclobutane pyrimidine dimers is an important component of UVB mutagenesis. Journal of Biological Chemistry (in press).
Markovitsi, D. (2016). UV-induced DNA damage: the role of electronic excited states. Photochemistry and Photobiology, 92(1), 45–51. https://doi.org/10.1111/php.12533.
Martinez-Fernandez, L., Banyasz, A., Esposito, L., Markovitsi, D., & Improta, R. (2017). UV-induced damage to DNA: effect of cytosine methylation on pyrimidine dimerization. Signal Transduction and Targeted Therapy, 2, 17021. https://doi.org/10.1038/sigtrans.2017.21.
Mitchell, D. L., & Fernandez, A. A. (2011). Different types of DNA damage play different roles in the etiology of sunlight-induced melanoma. Pigment Cell & Melanoma Research, 24(1), 119–124. https://doi.org/10.1111/j.1755-148X.2010.00789.x.
Ngo, T. T., et al. (2016). Effects of cytosine modifications on DNA flexibility and nucleosome mechanical stability. Nature Communications, 7, 10813. https://doi.org/10.1038/ncomms10813.
Pfeifer, G. P. (2020). Mechanisms of UV-induced mutations and skin cancer. Genome Instability & Disease, 1, 99–113.
Premi, S., & Brash, D. E. (2016). Chemical excitation of electrons: a dark path to melanoma. DNA Repair, 44, 169–177. https://doi.org/10.1016/j.dnarep.2016.05.023.
Rochette, P. J., et al. (2003). UVA-induced cyclobutane pyrimidine dimers form predominantly at thymine–thymine dipyrimidines and correlate with the mutation spectrum in rodent cells. Nucleic Acids Research, 31(11), 2786–2794.
Rochette, P. J., Lacoste, S., Therrien, J. P., Bastien, N., Brash, D. E., & Drouin, R. (2009). Influence of cytosine methylation on ultraviolet-induced cyclobutane pyrimidine dimer formation in genomic DNA. Mutation Research, 665(1–2), 7–13. https://doi.org/10.1016/j.mrfmmm.2009.02.008.
Sage, E., Lamolet, B., Brulay, E., Moustacchi, E., Chteauneuf, A., & Drobetsky, E. A. (1996). Mutagenic specificity of solar UV light in nucleotide excision repair-deficient rodent cells. Proceedings of the National academy of Sciences of the United States of America, 93, 176–180.
Schreier, W. J., Gilch, P., & Zinth, W. (2015). Early events of DNA photodamage. Annual Review of Physical Chemistry, 66, 497–519. https://doi.org/10.1146/annurev-physchem-040214-121821.
Setlow, R. B., & Setlow, J. K. (1962). Evidence that ultraviolet-induced thymine dimers in DNA cause biological damage. Proceedings of the National Academy of Sciences of the United States of America, 48, 1250–1257. https://doi.org/10.1073/pnas.48.7.1250.
Sharonov, A., Gustavsson, T., Marguet, S., & Markovitsi, D. (2003). Photophysical properties of 5-methylcytidine. Photochemical and Photobiological Sciences, 2(4), 362–364.
Shen, L., et al. (2013). Genome-wide analysis reveals TET- and TDG-dependent 5-methylcytosine oxidation dynamics. Cell, 153(3), 692–706. https://doi.org/10.1016/j.cell.2013.04.002.
Tahiliani, M., et al. (2009). Conversion of 5-methylcytosine to 5-hydroxymethylcytosine in mammalian DNA by MLL partner TET1. Science, 324(5929), 930–935.
Takaya, T., Su, C., de La Harpe, K., Crespo-Hernandez, C. E., & Kohler, B. (2008). UV excitation of single DNA and RNA strands produces high yields of exciplex states between two stacked bases. Proceedings of the National Academy of Sciences of the United States of America, 105(30), 10285–10290. https://doi.org/10.1073/pnas.0802079105.
Tommasi, S., Denissenko, M. F., & Pfeifer, G. P. (1997). Sunlight induces pyrimidine dimers preferentially at 5-methylcytosine bases. Cancer Research, 57, 4727–4730.
Tornaletti, S., & Pfeifer, G. P. (1995). Complete and tissue-independent methylation of CpG sites in the p53 gene: implications for mutations in human cancers. Oncogene, 10, 1493–1499.
Vendrell-Criado, V., Rodriguez-Muniz, G. M., Lhiaubet-Vallet, V., Cuquerella, M. C., & Miranda, M. A. (2016). The (6–4) dimeric lesion as a DNA photosensitizer. ChemPhysChem, 17(13), 1979–1982. https://doi.org/10.1002/cphc.201600154.
Vu, B., Cannistraro, V. J., Sun, L., & Taylor, J. S. (2006). DNA synthesis past a 5-methylC-containing cis-syn-cyclobutane pyrimidine dimer by yeast pol eta is highly nonmutagenic. Biochemistry, 45(30), 9327–9335.
Wacker, A., Dellweg, H., & Jacherts, D. (1962). Thymine dimerization and survival of bacteria. Journal of Molecular Biology, 4, 410–412. https://doi.org/10.1016/s0022-2836(62)80022-9.
Wang, X., et al. (2019). Ultrafast Intersystem Crossing in Epigenetic DNA Nucleoside 2’-Deoxy-5-formylcytidine. The Journal of Physical Chemistry B, 123(27), 5782–5790. https://doi.org/10.1021/acs.jpcb.9b04361.
Yasuda, S., & Sekiguchi, M. (1970). T4 endonuclease involved in repair of DNA. Proceedings of the National Academy of Sciences of the United States of America, 67(4), 1839–1845. https://doi.org/10.1073/pnas.67.4.1839.
Yoon, J.-H., Lee, C.-S., O’Connor, T., Yasui, A., & Pfeifer, G. P. (2000). The DNA damage spectrum produced by simulated sunlight. Journal of Molecular Biology, 299, 681–693.
You, Y. H., Lee, D. H., Yoon, J. H., Nakajima, S., Yasui, A., & Pfeifer, G. P. (2001). Cyclobutane pyrimidine dimers are responsible for the vast majority of mutations induced by UVB irradiation in mammalian cells. Journal of Biological Chemistry, 276, 44688–44694.
You, Y.-H., Li, C., & Pfeifer, G. P. (1999). Involvement of 5-methylcytosine in sunlight-induced mutagenesis. Journal of Molecular Biology, 293, 493–503.
You, Y. H., & Pfeifer, G. P. (2001). Similarities in sunlight-induced mutational spectra of CpG-methylated transgenes and the p53 gene in skin cancer point to an important role of 5-methylcytosine residues in solar UV mutagenesis. Journal of Molecular Biology, 305, 389–399.
You, Y. H., Szabo, P. E., & Pfeifer, G. P. (2000). Cyclobutane pyrimidine dimers form preferentially at the major p53 mutational hotspot in UVB-induced mouse skin tumors. Carcinogenesis, 21(11), 2113–2117. https://doi.org/10.1093/carcin/21.11.2113.
Acknowledgements
This study was supported by NIH grant R37 ES006070 to G.P.P.
Author information
Affiliations
Beckman Research Institute of the City of Hope, Duarte, USA
Sang-In Kim
Center for Epigenetics, Van Andel Institute, Grand Rapids, MI, USA
Gerd P. Pfeifer
Contributions
GPP designed the research. SIK performed the research. SIK and GPP analyzed the data. GPP wrote the manuscript.
Corresponding author
Correspondence to Gerd P. Pfeifer.
Ethics declarations
Conflict of interest
The authors declare that they have no conflict of interest.
Supplementary Information
Below is the link to the electronic supplementary material.
Supplementary file1 (PDF 4571 KB)
Rights and permissions
About this article
Cite this article
Kim, SI., Pfeifer, G.P. The epigenetic DNA modification 5-carboxylcytosine promotes high levels of cyclobutane pyrimidine dimer formation upon UVB irradiation. GENOME INSTAB. DIS. (2021). https://doi.org/10.1007/s42764-020-00030-x
Received11 September 2020
Revised24 October 2020
Accepted27 November 2020
Published02 January 2021
Share this article
Anyone you share the following link with will be able to read this content:
Get shareable linkKeywords
UVB
DNA damage
Cyclobutane pyrimidine dimer
5-carboxylcytosine
DNA methylation
用户登录
还没有账号?
立即注册