The post translational modification of key regulators of ATR signaling in DNA replication
Review Article
Genome Instability & Disease , 2 92–101(2021)
Abstract
DNA replication is one of the most critical psychological process, which mainly consists of three steps: initiation, elongation, and termination. Precise regulation through all stages of DNA replication is essential for maintaining genome integrity in proliferating cells. During each cell cycle, the complete genetic information existing in the DNA needs to be duplicated and passed on to the daughter cells. The DNA replication machinery is confronted with many challenges and stresses owing to endogenous and exogenous facts that could harm the faithful duplication of the DNA. The abnormality of DNA replication could cause genomic instability and tumorigenesis. ATR is a master regulator of cells in response to DNA replication stress to safeguard genomic stability and prevent tumorigenesis. In the past years, the key functions of post-translational modification (PTM) in ATR signaling transduction has been explored. ATR signaling is complicatedly and tightly regulated by multiple PTMs, such as phosphorylation, acetylation, ubiquitination, SUMOylation and so on. Here, we summarize how the ATR signaling pathway is tightly regulated by PTM. Furthermore, we describe the critical roles of ATR signaling in DNA replication and safeguarding genomic stability. In the past years, the key functions of post-translational modification (PTM) in DNA replication has been explored. These findings benefit for us better understand the complicated mechanism of DNA replication signaling pathway.
The compositions and work model of ATR signaling in DNA replication stress
ATR signaling pathway is consisted by several key proteins as shown in Table1 (Awasthi et al., 2015; Blackford & Jackson, 2017; Maréchal & Zou, 2013; Yang, 2004). ATR (ATM and rad3-related), the central regulator of ATR signaling pathway, is one member of PI3K-Related Kinases (PIKKs) families, which are mainly consisted of five domains: HEAT repeat, FAT domain, kinase domain, PIKK regulatory domain(PRD) and FACT domain, as shown in Fig. 1. Other members of the PIKK family contain ataxia telangiectasia mutated (ATM), DNA-dependent protein kinase (DNA–PK), homolog of Caenorhabditis elegans SMG-1 (SMG1) and mammalian target of rapamycin (mTOR,also known as FRAP). Since the PIKKs family share a highly conserved catalytic domain, their target motif is very similar. For example, ATR, ATM, SMG-1 and DNA-PK preferentially phosphorylate Ser and Thr residues followed by a Gln (S/T-Q motif) (Baretic, 2019; Cimprich & Cortez, 2008; Mordes et al., 2008). ATR is one of the most important master regulators in DNA replication, DNA repair, senescence and apoptosis. Compared to ATM, which is mainly activated by DNA double-strand breaks (DSBs), ATR is a much broader responder to various types of DNA damage, including DSBs and other multiple types of DNA damage that are implicated to DNA replication (Byun et al., 2005; Kumagai et al., 2004; Walter & Newport, 2000). It is found that the HEAT repeat domain of ATR is responsible for binding with ATRIP (ATR interacting protein, the partner of ATR) and the FAT domain that provides structural assistance to the kinase domain. The kinase domain contains PRD (PIKK regulatory domain), which is responsible with binding with ToPBP1 and FATC domain (Burrows & Elledge, 2008; Lovejoy & Cortez, 2009; Wang, 2017).
Table 1 The composition and function of ATR signaling pathwayFull size tableFig. 1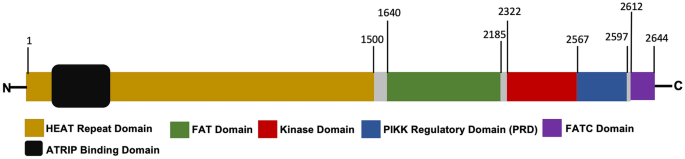
Schematic of the functional domains of ATR. The ATR (homo) is consisted of 2644 amino acids and primarily divided into 5 domains, including HEAT repeat domain, FAT domain, Kinase domain, PIKK regulatory domain (PRD) and FATC domain. The HEAT domain is important for ATR binding to ATRIP
Full size imageExtensive studies have suggested that ATR is activated by multiple types of DNA damage, including telomere deprotection, DSB or agents that involved in DNA replication (Maréchal & Zou, 2013; Matsuoka, 2007), as shown in Fig. 2. Firstly, DNA damage or stalled replication forks generated ssDNA that is coated and protect by RPA, and then RPA directly binds to ATRIP which is a partner of ATR and recruits it to DNA damage sites. Hence, ATR-ATRIP complex are recruited to ssDNA (Maréchal, 2014; Maréchal & Zou, 2015; Wu, 2014; Zou & Elledge, 2003). Secondly, TopBP1 which is composed of several BRCT repeats domain (9 in mammals and Xenopus, 4 in yeast) is essential to full activation of ATR–ATRIP (Lee et al. 2007; Mordes et al., 2008). The BRCT repeat domains play a key role in recognition of phosphorylated factors (Glover et al., 2004; Yu et al., 2003). Moreover, the region between BRCT6 and BRCT7 of TopBP1 is responsible for inducing the ATR-ATRIP activation (Ohashi et al., 2014; Wardlaw et al., 2014). Additionally, the specific DNA structure including dsDNA-ssDNA junction derived from stalled replication forks or DSBs resection also activates ATR signaling pathway (Ma et al., 2020; Shiotani, 2013). Except recruiting the ATR-ATRIP complex to the DNA damage sites, RPA also brings the clamp loader RAD17-RFC to the junctions (Lee & Dunphy, 2010; Yang & Zou, 2006). Further, RAD17-RFC recruits the RAD9-HUS1-RAD1 clamp (9-1-1) to the junctions (Navadgi-Patil & Burgers, 2011). The N-terminal domain of RAD9 combined with HUS1 and RAD1 forms a heterotrimer, forming a ring-shaped structure similar to PCNA (Delacroix et al., 2007; Friedrich-Heineken, 2005). The C-terminus of RAD9 is phosphorylated at Ser387 and creates a binding site for BRCT1-2 domain of TopBP1, which domain is essential for recruiting TopBP1 to dsDNA-ssDNA junctions. Finally, TopBP1 interacts with and activates ATR-ATRIP complexes, further leading to the activation and amplification of ATR-CHK1 signaling pathway (Day, 2018; Delacroix et al., 2007; Liu, 2014). In addition, it is reported that ETAA1, an RPA binding protein, is able to directly activate ATR independently of TopBP1 (Haahr, 2016). It is shown that CLASPIN functions as an adaptor between ATR-IP and CHK1, and works as a key regulator involving in ATR mediated phosphorylation and activation of CHK1 (Kumagai & Dunphy, 2000; Liu, 2006). Once activated, ATR phosphorylates multiple downstream effects, especially CHK1, facilitating ATR-CHK1 pathway to exert several key functions to promote replication fork stability and prevent genome instability.
Fig. 2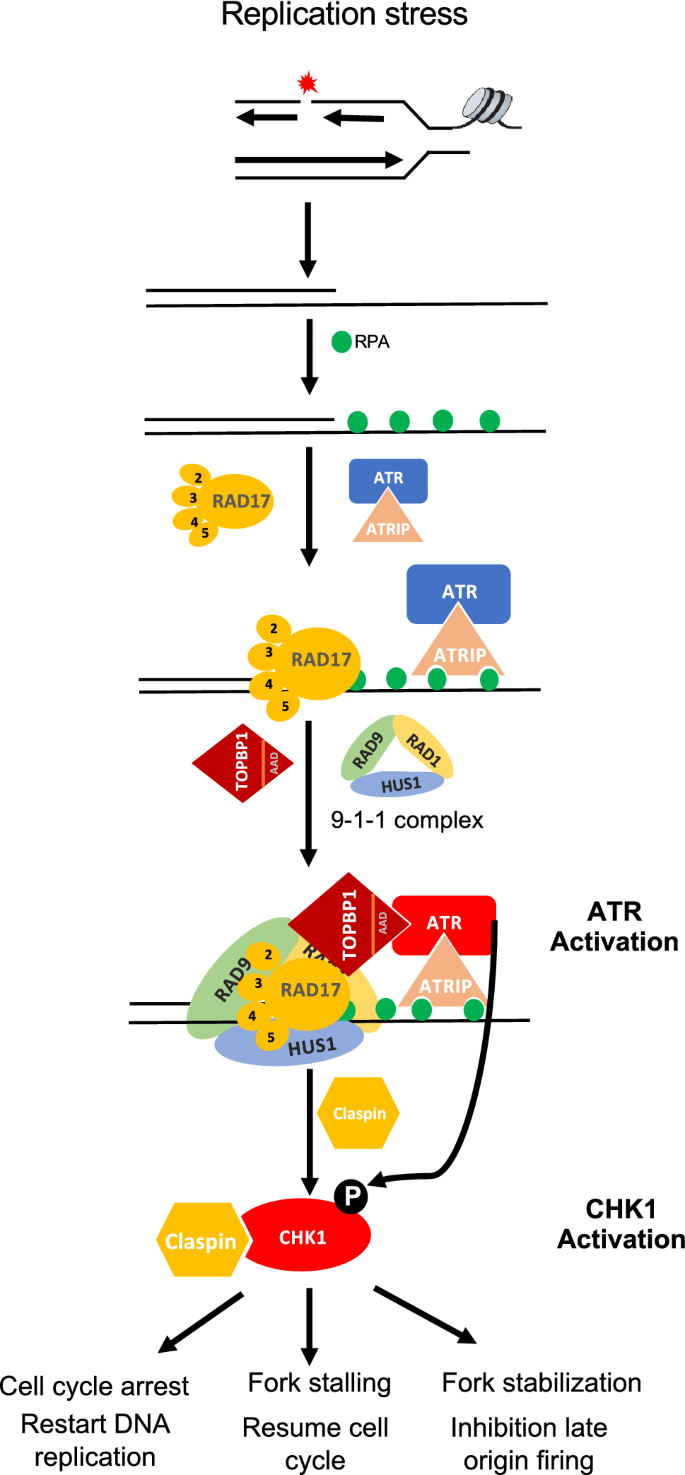
Model of ATR-CHK1 pathway activation by primed ssDNA during replication stress. ATR-CHK1 pathway is activated in a multi-step and multi-protein participation process. Firstly, RPA binds to and protect ssDNA. Then, ATR-ATRIP is recruited to ssDNA by RPA. Besides, RPA also promotes the recruitment of RAD17-Rfc2-5 clamp loader to junctions between ssDNA and dsDNA and the loading of 9-1-1 complex (RAD9-RAD1-HUS1) checkpoint onto dsDNA. Further, TOPBP1 is recruited to dsDNA by 9-1-1 complex. Then, ATR is activated by TOPBP1 via its ATR activation domain (AAD). Red color represents ATR activation status. The activated ATR further phosphorylates and activates CHK1 with the help of Claspin, resulting in ATR-CHK1 pathway activation to execute multiple functions to promote replication fork stability and so on
Full size imageThe ubiquitination and deubiquitination in ATR-CHK1 signaling pathway
Ubiquitination is one of extensively investigated PTMs, which are involved in regulating multiple physiological processes in eukaryotes, including DNA damage response, proliferation, tumorigenesis and cell cycle (Mansour, 2018; Ulrich & Walden, 2010). This PTM is usually mediated by three sequential steps: activation, conjugation, and ligation, which are catalyzed by ubiquitin-activating enzyme (E1), ubiquitin-conjugating enzyme (E2), and ubiquitin ligase (E3) enzymes, respectively. Ubiquitin, a highly conserved small protein composed of 76 amino acids, is covalently attached to lysine residues of substrate proteins (Mansour, 2018). Ubiquitination is firstly identified as a signal to induce substrates degradation by ubiquitin-proteasomal degradation system. Except for the protein degradation, ubiquitination also plays a key role in signal transduction (Swatek & Komander, 2016; Wilkinson, 2000). Ubiquitination is reported to regulate ATR-CHK1 signaling pathway involving in DNA replication (Cartel & Didier, 2020; Shiotani & Zou, 2009; Yu et al., 2020; Bennett & Clarke, 2006; Ghosh & Saha, 2012; Mirsanaye et al., 2021).
RPA, a key player in ATR activation, is ubiquitinated by PRP19 and RFWD3, resulting in promoting ATR activation and homologous recombination repair (HR) upon DNA damage (Dubois, 2017; Elia, 2015). In addition, RPA ubiquitinated by RFWD3 also facilitates the removal of RPA from DNA damage site and repair at Stalled Replication Forks (Elia, 2015; Inano, 2017). The E3 ligase, HUWE1 was reported to mediate ubiquitination of CHK1 and induce its degradation by ubiquitin–proteasome system (Cassidy et al., 2020). Moreover, two Cullin RING Ligase (CRL) complexes, E3 ligases regulate the CHK1 protein level, including the SKP1-Cullin1(Cul1)-Fbx complex, which mainly ubiquitylates CHK1 in the nucleus, whereas the CDT2-Cullin4A(Cul4A)-DDB1 complex primarily ubiquitylates CHK1 in the cytoplasm during replication stress (Cartel & Didier, 2020; Cassidy et al., 2020; Zheng et al., 2016). Meanwhile, TRAF4-mediated ubiquitination of CHK1 at K132 is essential for CHK1 phosphorylation and is activated by ATR (Yu, 2020). Moreover, BTG3-mediated CHK1 K63-linked ubiquitination regulates the chromatin loading and activity of CHK1 (Cheng et al., 2013). Interestingly, HDAC6, a member of Histone deacetylases (HDAC), potentiates E3 ligase activity and is able to promote CHK1 ubiquitination, resulting in its degradation (Moses, 2020).
The E3 ligase, HERC2 is a component of the DNA replication fork complex (Izawa, 2011). It participates in regulating replication stress by USP20-CLASPIN axis (Yuan, 2014; Zhu et al., 2014). Moreover, HERC2-mediated ubiquitination of RPA regulates its phosphorylation by ATR and ubiquitin-proteasomal degradation (Wu, 2018). Ubiquitination of CLASPIN by BRCA1 regulates its degradation upon topoisomerase 1 inhibition (Sato, 2012). APC/C Cdh1 system-mediated polyubiquitylation of CLASPIN facilitates its degradation in G1 phase (Bassermann, 2008). In addition, PLK1-mediated phosphorylation of CLASPIN promotes its ubiquitination by SCF-bTrCP ubiquitin ligase, resulting in its proteasome-dependent degradation (Peschiaroli, 2006). In addition, Cdh1/APC system mediates Rad17 degradation via ubiquitin–proteasome causing the termination of checkpoint signaling and the recovery from genotoxic stress (Zhang, 2010). 9-1-1 complex is monoubiquitinated at Lys197 of the Rad9 subunit by the Rad6/Rad18 complex, which in turn facilitates the activation of 9-1-1 complex after DNA damage (Fu, 2008). hHYD (also known as UBR5), a HECT-domain ubiquitin ligase, binds with TopBP1 via its BRCT domain and ubiquitinates TopBP1, resulting in TopBP1 instability. Moreover, upon DNA damage, TopBP1 is phosphorylated, which in turn inhibits its ubiquitination (Honda, 2002).
Deubiquitination is the reverse reaction of ubiquitination, is mediated by multiple deubiquitinases, which are responsible for removing ubiquitin from the substrates, and plays a vital role in the regulation of protein stability, activity, and its subcellular localization (Farshi, 2015; Reyes-Turcu et al., 2009; Sun, Liu, et al., 2020). So far, there has been about 80 deubiqutinases identified and mainly classified in 5 distinct families, including ubiquitin-specific proteases (USPs), ubiquitin C-terminal hydrolases (UCHs), ovarian tumor proteases (OTUs), Machado–Joseph disease (MJD) protein domain proteases, and JAMM motif zinc metalloproteases (Sun, Shi, et al., 2020; Young et al., 2019). Similar to ubiquitination, deubiquitination regulates various cellular processes, including DNA damage response, cell cycle, apoptosis, DNA replication, and tumorigenesis (Brinkmann et al., 2015).
CLASPIN is a key player in ATR-CHK1 signaling and DNA replication, and is regulated by several deubiquitinases. USP28 antagonizes the APC/C Cdh1-mediated degradation of CLASPIN (Zhang et al., 2006). Additionally, USP7 and USP29 stabilize CLASPIN and reverse its degradation mediated by SCF-bTrCP (Faustrup et al., 2009; Martín, 2015). Intriguingly, our previous study and Xu lab’s work also uncovered that USP20 functions as a DUB for CLASPIN, maintaining its stability upon replication stress (Yuan, 2014; Zhu et al., 2014). USP9X deubiquitinates and stabilizes CLASPIN in an S-phase-specific manner and maintains genomic stability during DNA replication (McGarry, 2016). Intriguingly, USP20 also deubiquitinates and stabilizes Rad17 following DNA damage, resulting in replication fork stability (Shanmugam, 2014).
CHK1 is regulated by multiple deubiquitinases. USP3 removes the K63-linked ubiquitination from CHK1, which facilitates its release from chromatin. USP37 could cleave the poly-ubiquitination chains of CHK1 and increase CHK1 protein stability, which in turn facilitates cellular response to replication stress (2019). Interestingly, in addition to CLAPIN, USP7 deubiquitinates and stabilizes CHK1 (Alonso-de Vega et al., 2014). Even though RPA and 9-1-1 were reported to be ubiquitinated as mentioned above, the deubiquitinases for them remain to be explored. Taken together, the ubiquitination and deubiquitination of key regulators in the ATR-CHK1 pathway and have vital roles in the regulation of ATR signaling transduction and cellular response to DNA replication stress.
The phosphorylation in ATR-CHK1 signaling pathway
Phosphorylation, which is the most-explored PTM, plays key roles in regulating various physiological processes, including enzymatic activities, protein localization, DNA replication, gene expression, DNA damage response, and signal transduction (Ardito et al., 2017). ATR, the master of ATR-CHK1 signaling, is a kinase (Nam & Cortez, 2011). Autophosphorylation is essential for ATR activation and ATR signaling transduction, which plays an important role in constructing of a signal network in DNA replication stress to maintain replication fork stability.
Upon replication stress, RPA-coated ssDNA recruits ATR-ATRIP complex to sites of DNA damage and initiates ATR activation. Besides that, the full activation of ATR needs to trigger autophosphorylation at Thr1989 which creates a binding site for BRCT domains 7 and 8 of TopBP1 and promoting ATR substrate recognition (Liu, 2011; Nam, 2011). Hence, ATR autophosphorylation is crucial for its full activation. CHK1, a well-known substrate of ATR, is not only a key player for the checkpoint response, but also vital for the stability of DNA replication forks. Upon DNA damage, CHK1 is phosphorylated by ATR at Ser317 and Ser345 and activated to regulate cell cycle progression, maintain replication fork stability and promote faithful chromosome segregation (Goto et al., 2015; Kabeche et al., 2018). In addition, CLASPIN is phosphorylated at Thr916 and Ser945 in response to DNA replication stress, which is possibly mediated by ATR (Bennett et al., 2008). The phosphorylation is crucial to establish binding between CLAPIN and CHK1 (Bennett et al., 2008; Clarke & Clarke, 2005). Besides that, CDK7-mediated phosphorylation of CLASPIN is essential for the activation of the ATR–Chk1 signaling pathway (Yang et al., 2019).
RPA is a heterotrimeric complex composed of three subunits, RPA70, RPA32, and RPA14 (Feldkamp et al., 2014). Accumulating research has shown that the phosphorylation of RPA32 plays a vital role in RPA complex functions. RPA32 is phosphorylated on multiple N-terminal residues during replication stress (Oakley, 2009). Unperturbed, RPA32 participates in the regulation of mitosis and G1/S phase transition via its phosphorylation by cyclin-dependent kinase 1 (CDK1)/cyclin B and CDK2/cyclin A, respectively (Anantha et al., 2007). Moreover, ATR phosphorylates RPA32 at Ser33 in response to DNA damage and replication stress, which is the key and initial step for subsequent phosphorylation at Ser29 and Ser4/Ser8 phosphorylation by DNA-PKcs (Ashley, 2014; Glanzer, 2014; Shiotani, 2013).
Interestingly, ATRIP, the partner of ATR, is phosphorylated at Ser68 and Ser72 by ATR in response to genotoxic stimuli (Itakura, 2004). But, the phosphorylation is dispensable for ATR-mediated signaling. Rad9, a key component of heterotrimeric complex (the 9-1-1 complex), is phosphorylated at Ser272 by ATR and ATM in response to DNA damage, which is indispensable for CHK1 activation (Roos-Mattjus, 2002, 2003). Moreover, the Tousled-like kinase 1B (TLK1B) indirectly phosphorylates Rad9 at S328, which causes its release from stalled replication forks, leading to the instability of stalled replication forks and prolonged activation of the S-phase checkpoint (Benedetti, 2012). Rad17, a substrate of ATR, is phosphorylated at Ser635 and Ser645 upon replication interference, which is essential for ATR signaling and replication (Post, 2001; Wang, 2006). TopBP1 is directly phosphorylated by ATM at several residues, including, Ser405, Ser476, Ser1051, Ser766 and Thr975, which phosphorylation is required for cell survival in response to replication stress (Yamane et al., 2002).
The acetylation and deacetylation in ATR-CHK1 signaling pathway
Acetylation is a reversible and dynamic process and is controlled by several deacetylases and acetyltransferases. Accumulating research has shown acetylation is an important PTM exerting vital function in multiple processes, including DNA damage response, replication stress, cell cycle checkpoint, and transcription (Glozak et al., 2005; Xia et al., 2020). p300, an acetyltransferase, acetylates RPA resulting in increasing its binding affinity to ssDNA (Surendran et al., 2016). p300 is also reported as an acetyltransferase for TopBP1, which regulates its activity and function (Liu, 2014). Moreover, acetyltransferases, GCN5 and PCAF mediated RPA70 acetylation at lysine 163, which is important for nucleotide excision repair (NER) in response to UV irradiation (He et al., 2017).
Sirtuin 2 (SIRT2) interacts with and deacetylates ATRIP at Lys32 in response to replication stress, which in turn facilitates the recruitment of ATRIP to chromatin and ATR activation (Zhang, 2016). SIRT1, the mammalian homolog of yeast Sir2, is a member of the sirtuin family of type III histone deacetylases and deacetylates TopBP1, which in turn participates in regulation of DNA replication fork initiation and the intra-S-phase cell cycle checkpoint (Wang, 2014). On the other hand, Sirt1 deacetylates TopBP1 upon glucose deprivation, resulting in TopBP1-Treslin disassociation and DNA replication inhibition (Liu, 2014). In contrast, SIRT1 activity is inhibited upon genotoxic stress, resulting in an increase in TopBP1 acetylation, which is vital for the TopBP1-Rad9 interaction and activation of the ATR-Chk1 pathway. Mechanistically, the acetylation of TopBP1 induces its conformation change, which promotes its interaction with distinct partners in DNA replication and checkpoint activation (Liu, 2014). HDAC6, a member of Histone deacetylases (HDACs), antagonize GCN5 and PCAF mediated RPA70 acetylation (Zhao, 2017).
The SUMOylation and deSUMOylation in ATR-CHK1 signaling pathway
SUMOylation is achieved via the small ubiquitin-like modifier (SUMO) family of proteins. SUMO is conjugated to lysine residues of substrates by SUMO-specific E1, E2, and E3 (Yang, 2017). Like deubiquitination, SUMOylation is also a reversible dynamic process. The SUMO modification is removed by sentrin/SUMO-specific proteases (SENPs), which removal reaction is called deSUMOylation (Nayak & Müller, 2014). SUMOylation plays a vital role in regulating multiple process, including protein localization, interaction, transcription, DNA repair, and DNA replication (Celen & Sahin, 2020).
ATRIP is SUMOylated at K234 and K289, which SUMOylation facilitates its binding to multiple protein including ATR, RPA70, TopBP1, and the MRE11–RAD50–NBS1 complex (Wu, 2014; Zhou, 2020). Moreover, RPA70 is SUMOylated at Lys449 and Lys577 under replication-mediated DSBs, which results in recruitment of Rad51 to promote DNA damage repair (Dou et al., 2010). In addition, TopBP1 is highly SUMOylated in response to DNA inter-strand crosslinking damage, which SUMOylation is important in regulation of replication stress and prohibiting fork breakage to protect DNA integrity (Munk, 2017).
SENP6, a sentrin/SUMO-specific protease (SENP), is responsible for removing SUMOs from RPA70 (Li, 2018). In addition, SENP5 is able to bind to ATRIP and facilitate ATRIP deSUMOylation (Jin et al., 2016).
The methylation and crotonylation in ATR-CHK1 signaling pathway
Accumulating studies suggest that the methylation of non-histone proteins has emerged as extensive PTM, which plays as a vital regulator of multiple cellular processes, including cell survival, DNA damage response, transcription, and DNA replication (Blasi, 2021; Hamamoto et al., 2015; Rodríguez-Paredes & Lyko, 2019). Rad9 is methylated by protein arginine methyltransferase 5 (PRMT5), which plays a critical role in regulation of Chk1 activation and maintaining genome integrity (He, 2011). Crotonylation, a novel PTM, is discovered in recent year (Wan et al., 2019). RPA70 is reported to be crotonylated at Lys88, Lys379 and Lys595 in response to DNA damage, which in turn promotes its bind with ssDNA and HR factors (Yu, 2020).
Conclusions
Extensive and inspiring research of PTM of the key regulators in ATR signaling have been published (Yazinski & Zou, 2016). Herein, we simply summarized and listed ubiquitination, phosphorylation, acetylation, methylation, crotonylation, and some of their inverse processes, which are related to ATR pathway and DNA replication stress. We hope these will be benefit for us to understand the progress of this field. At the same time, we are looking forward to more in-depth and systematic research in this field since there are still a lot of questions remaining to be answered. For example, as we know most of the PTMs are a reversible and dynamic processes, however, some of these reverse processes function in DNA replication stress are still unclear. Hence, further studies need to be carried out to focusing on these regulations. In addition, these PTMs occurring seem to be spatiotemporal specific and need to be very accurate (Dantuma & Attikum, 2016), so how do cells coordinate these regulations and how they work together to make cells better cope with the DNA replication stress and safeguard genomic integrity? Further efforts need to be done to clarify the interplay among these modifiers and their contribution during DNA replication stress.
Since ATR-CHK1 signaling plays an important role in maintaining genome stability, prevent tumorigenesis and chemo/radio-resistance (Smith et al. 2010; Yazinski & Zou, 2016), targeting ATR-CHK1 signaling has been identified as an effective approach to kill cancer (Primo & Teixeira, 2019; Sørensen & Syljuåsen, 2012; Wilhelm et al., 2020). Several CHK1 inhibitors and ATR inhibitors have been developed to treat cancer (Heo, 2019; Rundle et al., 2017). Better understanding the molecular mechanism of activation of ATR-CHK1 signaling would be of valuable importance for exploring novel therapeutic target. With the study progress of PTMs of ATR signaling, it provides additional choices and targets for cancer therapy as well as biomarkers for cancer diagnosis. In addition to targeting these key components of ATR-CHK1 pathway themselves, we can also target these key proteins that mediate PTMs of ATR signaling. Inspiringly, owing to great progress had achieved in technologies and other tools, including bioinformatics workflow and proteomics, the possibility is increases to identify not only novel PTMs but also the known PTMs on previously unrecognized modified proteins in ATR signaling pathway, which will inspire us to identify novel target for disease related to aberrant regulation of DNA replication and ATR pathway.
References
Alonso-de Vega, I., Martín, Y., & Smits, V. A. (2014). USP7 controls Chk1 protein stability by direct deubiquitination. Cell Cycle (Georgetown, Tex.), 13, 3921–3926. https://doi.org/10.4161/15384101.2014.973324
Anantha, R. W., Vassin, V. M., & Borowiec, J. A. (2007). Sequential and synergistic modification of human RPA stimulates chromosomal DNA repair. The Journal of Biological Chemistry, 282, 35910–35923. https://doi.org/10.1074/jbc.M704645200
Ardito, F., Giuliani, M., Perrone, D., Troiano, G., & Lo Muzio, L. (2017). The crucial role of protein phosphorylation in cell signaling and its use as targeted therapy (Review). International Journal of Molecular Medicine, 40, 271–280. https://doi.org/10.3892/ijmm.2017.3036
Ashley, A. K., et al. (2014). DNA-PK phosphorylation of RPA32 Ser4/Ser8 regulates replication stress checkpoint activation, fork restart, homologous recombination and mitotic catastrophe. DNA Repair, 21, 131–139. https://doi.org/10.1016/j.dnarep.2014.04.008
Awasthi, P., Foiani, M., & Kumar, A. (2015). ATM and ATR signaling at a glance. Journal of Cell Science, 128, 4255–4262. https://doi.org/10.1242/jcs.169730
Baretic, D., et al. (2019). Structural insights into the critical DNA damage sensors DNA-PKcs, ATM and ATR. Progress in Biophysics and Molecular Biology, 147, 4–16. https://doi.org/10.1016/j.pbiomolbio.2019.06.003
Bassermann, F., et al. (2008). The Cdc14B-Cdh1-Plk1 axis controls the G2 DNA-damage-response checkpoint. Cell, 134, 256–267. https://doi.org/10.1016/j.cell.2008.05.043
Bennett, L. N. & Clarke, P. R. (2006). Regulation of Claspin degradation by the ubiquitin-proteosome pathway during the cell cycle and in response to ATR-dependent checkpoint activation. FEBS Letters, 580, 4176–4181. https://doi.org/10.1016/j.febslet.2006.06.071
Bennett, L. N., Larkin, C., Gillespie, D. A., & Clarke, P. R. (2008). Claspin is phosphorylated in the Chk1-binding domain by a kinase distinct from Chk1. Biochemical and Biophysical Research Communications, 369, 973–976. https://doi.org/10.1016/j.bbrc.2008.02.154
Blackford, A. N., & Jackson, S. P. (2017). ATM, ATR, and DNA-PK: The trinity at the heart of the DNA damage response. Molecular Cell, 66, 801–817. https://doi.org/10.1016/j.molcel.2017.05.015
Brinkmann, K., Schell, M., Hoppe, T., & Kashkar, H. (2015). Regulation of the DNA damage response by ubiquitin conjugation. Frontiers in Genetics, 6, 98. https://doi.org/10.3389/fgene.2015.00098
Burrows, A. E., & Elledge, S. J. (2008). How ATR turns on: TopBP1 goes on ATRIP with ATR. Genes & Development, 22, 1416–1421. https://doi.org/10.1101/gad.1685108
Byun, T. S., Pacek, M., Yee, M. C., Walter, J. C., & Cimprich, K. A. (2005). Functional uncoupling of MCM helicase and DNA polymerase activities activates the ATR-dependent checkpoint. Genes & Development, 19, 1040–1052. https://doi.org/10.1101/gad.1301205
Cartel, M., & Didier, C. (2020). Regulation of CHK1 by the ubiquitin-proteasome system. The FEBS Journal, 287, 1982–1984. https://doi.org/10.1111/febs.15173
Cassidy, K. B., Bang, S., Kurokawa, M., & Gerber, S. A. (2020). Direct regulation of Chk1 protein stability by E3 ubiquitin ligase HUWE1. The FEBS Journal, 287, 1985–1999. https://doi.org/10.1111/febs.15132
Celen, A. B., & Sahin, U. (2020). Sumoylation on its 25th anniversary: mechanisms, pathology, and emerging concepts. The FEBS Journal, 287, 3110–3140. https://doi.org/10.1111/febs.15319
Cheng, Y. C., Lin, T. Y., & Shieh, S. Y. (2013). Candidate tumor suppressor BTG3 maintains genomic stability by promoting Lys63-linked ubiquitination and activation of the checkpoint kinase CHK1. Proceedings of the National Academy of Sciences of the United States of America, 110, 5993–5998. https://doi.org/10.1073/pnas.1220635110
Cimprich, K. A., & Cortez, D. (2008). ATR: An essential regulator of genome integrity. Nature Reviews Molecular Cell Biology, 9, 616–627. https://doi.org/10.1038/nrm2450
Clarke, C. A., & Clarke, P. R. (2005). DNA-dependent phosphorylation of Chk1 and Claspin in a human cell-free system. The Biochemical Journal, 388, 705–712. https://doi.org/10.1042/bj20041966
Dantuma, N. P., & van Attikum, H. (2016). Spatiotemporal regulation of posttranslational modifications in the DNA damage response. The EMBO Journal, 35, 6–23. https://doi.org/10.15252/embj.201592595
Day, M., et al. (2018). BRCT domains of the DNA damage checkpoint proteins TOPBP1/Rad4 display distinct specificities for phosphopeptide ligands. eLife. https://doi.org/10.7554/eLife.39979
De Benedetti, A. (2012). The tousled-like kinases as guardians of genome integrity. ISRN Molecular Biology, 2012, 627596. https://doi.org/10.5402/2012/627596
Delacroix, S., Wagner, J. M., Kobayashi, M., Yamamoto, K., & Karnitz, L. M. (2007). The Rad9-Hus1-Rad1 (9-1-1) clamp activates checkpoint signaling via TopBP1. Genes & Development, 21, 1472–1477. https://doi.org/10.1101/gad.1547007
Di Blasi, R., et al. (2021). Non-histone protein methylation: biological significance and bioengineering potential. ACS Chemical Biology. https://doi.org/10.1021/acschembio.0c00771
Dou, H., Huang, C., Singh, M., Carpenter, P. B., & Yeh, E. T. (2010). Regulation of DNA repair through deSUMOylation and SUMOylation of replication protein A complex. Molecular Cell, 39, 333–345. https://doi.org/10.1016/j.molcel.2010.07.021
Dubois, J. C., et al. (2017). A phosphorylation-and-ubiquitylation circuitry driving ATR activation and homologous recombination. Nucleic Acids Research, 45, 8859–8872. https://doi.org/10.1093/nar/gkx571
Elia, A. E., et al. (2015). RFWD3-dependent ubiquitination of RPA regulates repair at stalled replication forks. Molecular Cell, 60, 280–293. https://doi.org/10.1016/j.molcel.2015.09.011
Farshi, P., et al. (2015). Deubiquitinases (DUBs) and DUB inhibitors: A patent review. Expert Opinion on Therapeutic Patents, 25, 1191–1208. https://doi.org/10.1517/13543776.2015.1056737
Faustrup, H., Bekker-Jensen, S., Bartek, J., Lukas, J., & Mailand, N. (2009). USP7 counteracts SCFbetaTrCP- but not APCCdh1-mediated proteolysis of Claspin. The Journal of Cell Biology, 184, 13–19. https://doi.org/10.1083/jcb.200807137
Feldkamp, M. D., Mason, A. C., Eichman, B. F., & Chazin, W. J. (2014). Structural analysis of replication protein A recruitment of the DNA damage response protein SMARCAL1. Biochemistry, 53, 3052–3061. https://doi.org/10.1021/bi500252w
Friedrich-Heineken, E., et al. (2005). The two DNA clamps Rad9/Rad1/Hus1 complex and proliferating cell nuclear antigen differentially regulate flap endonuclease 1 activity. Journal of Molecular Biology, 353, 980–989. https://doi.org/10.1016/j.jmb.2005.09.018
Fu, Y., et al. (2008). Rad6-Rad18 mediates a eukaryotic SOS response by ubiquitinating the 9-1-1 checkpoint clamp. Cell, 133, 601–611. https://doi.org/10.1016/j.cell.2008.02.050
Ghosh, S. & Saha, T. (2012). Central role of ubiquitination in genome maintenance: DNA replication and damage repair. ISRN Molecular Biology, 2012, 146748. https://doi.org/10.5402/2012/146748
Glanzer, J. G., et al. (2014). RPA inhibition increases replication stress and suppresses tumor growth. Cancer Research, 74, 5165–5172. https://doi.org/10.1158/0008-5472.Can-14-0306
Glover, J. N., Williams, R. S., & Lee, M. S. (2004). Interactions between BRCT repeats and phosphoproteins: Tangled up in two. Trends in Biochemical Sciences, 29, 579–585. https://doi.org/10.1016/j.tibs.2004.09.010
Glozak, M. A., Sengupta, N., Zhang, X., & Seto, E. (2005). Acetylation and deacetylation of non-histone proteins. Gene, 363, 15–23. https://doi.org/10.1016/j.gene.2005.09.010
Goto, H., Kasahara, K., & Inagaki, M. (2015). Novel insights into Chk1 regulation by phosphorylation. Cell Structure and Function, 40, 43–50. https://doi.org/10.1247/csf.14017
Haahr, P., et al. (2016). Activation of the ATR kinase by the RPA-binding protein ETAA1. Nature Cell Biology, 18, 1196–1207. https://doi.org/10.1038/ncb3422
Hamamoto, R., Saloura, V., & Nakamura, Y. (2015). Critical roles of non-histone protein lysine methylation in human tumorigenesis. Nature Reviews. Cancer, 15, 110–124. https://doi.org/10.1038/nrc3884
He, H., Wang, J., & Liu, T. (2017). UV-Induced RPA1 acetylation promotes nucleotide excision repair. Cell Reports, 20, 2010–2025. https://doi.org/10.1016/j.celrep.2017.08.016
He, W., et al. (2011). A role for the arginine methylation of Rad9 in checkpoint control and cellular sensitivity to DNA damage. Nucleic Acids Research, 39, 4719–4727. https://doi.org/10.1093/nar/gkq1264
Heo, K. S. (2019). Regulation of post-translational modification in breast cancer treatment. BMB Reports, 52, 113–118. https://doi.org/10.5483/BMBRep.2019.52.2.017
Honda, Y., et al. (2002). Cooperation of HECT-domain ubiquitin ligase hHYD and DNA topoisomerase II-binding protein for DNA damage response. The Journal of Biological Chemistry, 277, 3599–3605. https://doi.org/10.1074/jbc.M104347200
Inano, S., et al. (2017). RFWD3-mediated ubiquitination promotes timely removal of both RPA and RAD51 from DNA damage sites to facilitate homologous recombination. Molecular Cell, 66, 622-634.e628. https://doi.org/10.1016/j.molcel.2017.04.022
Itakura, E., et al. (2004). ATR-dependent phosphorylation of ATRIP in response to genotoxic stress. Biochemical and Biophysical Research Communications, 323, 1197–1202. https://doi.org/10.1016/j.bbrc.2004.08.228
Izawa, N., et al. (2011). HERC2 Interacts with Claspin and regulates DNA origin firing and replication fork progression. Cancer Research, 71, 5621–5625. https://doi.org/10.1158/0008-5472.Can-11-0385
Jin, Z. L., Pei, H., Xu, Y. H., Yu, J., & Deng, T. (2016). The SUMO-specific protease SENP5 controls DNA damage response and promotes tumorigenesis in hepatocellular carcinoma. European Review for Medical and Pharmacological Sciences, 20, 3566–3573.
Kabeche, L., Nguyen, H. D., Buisson, R., & Zou, L. (2018). A mitosis-specific and R loop-driven ATR pathway promotes faithful chromosome segregation. Science (New York, N.Y.), 359, 108–114. https://doi.org/10.1126/science.aan6490
Kumagai, A., & Dunphy, W. G. (2000). Claspin, a novel protein required for the activation of Chk1 during a DNA replication checkpoint response in Xenopus egg extracts. Molecular Cell, 6, 839–849. https://doi.org/10.1016/s1097-2765(05)00092-4
Kumagai, A., Kim, S. M., & Dunphy, W. G. (2004). Claspin and the activated form of ATR-ATRIP collaborate in the activation of Chk1. The Journal of Biological Chemistry, 279, 49599–49608. https://doi.org/10.1074/jbc.M408353200
Lee, J., & Dunphy, W. G. (2010). Rad17 plays a central role in establishment of the interaction between TopBP1 and the Rad9-Hus1-Rad1 complex at stalled replication forks. Molecular Biology of the Cell, 21, 926–935. https://doi.org/10.1091/mbc.e09-11-0958
Lee, J., Kumagai, A., & Dunphy, W. G. (2007). The Rad9-Hus1-Rad1 checkpoint clamp regulates interaction of TopBP1 with ATR. The Journal of Biological Chemistry, 282, 28036–28044. https://doi.org/10.1074/jbc.M704635200
Li, J., et al. (2018). Desumoylase SENP6 maintains osteochondroprogenitor homeostasis by suppressing the p53 pathway. Nature Communications, 9, 143. https://doi.org/10.1038/s41467-017-02413-3
Liu, S., et al. (2006). Claspin operates downstream of TopBP1 to direct ATR signaling towards Chk1 activation. Molecular and Cellular Biology, 26, 6056–6064. https://doi.org/10.1128/mcb.00492-06
Liu, S., et al. (2011). ATR autophosphorylation as a molecular switch for checkpoint activation. Molecular Cell, 43, 192–202. https://doi.org/10.1016/j.molcel.2011.06.019
Liu, T., et al. (2014). A divergent role of the SIRT1-TopBP1 axis in regulating metabolic checkpoint and DNA damage checkpoint. Molecular Cell, 56, 681–695. https://doi.org/10.1016/j.molcel.2014.10.007
Lovejoy, C. A., & Cortez, D. (2009). Common mechanisms of PIKK regulation. DNA Repair, 8, 1004–1008. https://doi.org/10.1016/j.dnarep.2009.04.006
Ma, M., Rodriguez, A., & Sugimoto, K. (2020). Activation of ATR-related protein kinase upon DNA damage recognition. Current Genetics, 66, 327–333. https://doi.org/10.1007/s00294-019-01039-w
Mansour, M. A. (2018). Ubiquitination: Friend and foe in cancer. The International Journal of Biochemistry & Cell Biology, 101, 80–93. https://doi.org/10.1016/j.biocel.2018.06.001
Maréchal, A., et al. (2014). PRP19 transforms into a sensor of RPA-ssDNA after DNA damage and drives ATR activation via a ubiquitin-mediated circuitry. Molecular Cell, 53, 235–246. https://doi.org/10.1016/j.molcel.2013.11.002
Maréchal, A., & Zou, L. (2013). DNA damage sensing by the ATM and ATR kinases. Cold Spring Harbor Perspectives in Biology. https://doi.org/10.1101/cshperspect.a012716
Maréchal, A., & Zou, L. (2015). RPA-coated single-stranded DNA as a platform for post-translational modifications in the DNA damage response. Cell Research, 25, 9–23. https://doi.org/10.1038/cr.2014.147
Martín, Y., et al. (2015). USP29 controls the stability of checkpoint adaptor Claspin by deubiquitination. Oncogene, 34, 1058–1063. https://doi.org/10.1038/onc.2014.38
Matsuoka, S., et al. (2007). ATM and ATR substrate analysis reveals extensive protein networks responsive to DNA damage. Science (New York, N.Y.), 316, 1160–1166. https://doi.org/10.1126/science.1140321
McGarry, E., et al. (2016). The Deubiquitinase USP9X maintains DNA replication fork stability and DNA damage checkpoint responses by regulating CLASPIN during S-phase. Cancer Research, 76, 2384–2393. https://doi.org/10.1158/0008-5472.Can-15-2890
Mirsanaye, A. S., Typas, D. & Mailand, N. (2021). Ubiquitylation at stressed replication forks: mechanisms and functions. Trends in Cell Biology. https://doi.org/10.1016/j.tcb.2021.01.008
Mordes, D. A., Glick, G. G., Zhao, R., & Cortez, D. (2008). TopBP1 activates ATR through ATRIP and a PIKK regulatory domain. Genes & Development, 22, 1478–1489. https://doi.org/10.1101/gad.1666208
Moses, N., et al. (2020). HDAC6 regulates radiosensitivity of non-small cell lung cancer by promoting degradation of Chk1. Cells. https://doi.org/10.3390/cells9102237
Munk, S., et al. (2017). Proteomics reveals global regulation of protein SUMOylation by ATM and ATR kinases during replication stress. Cell Reports, 21, 546–558. https://doi.org/10.1016/j.celrep.2017.09.059
Nam, E. A., et al. (2011). Thr-1989 phosphorylation is a marker of active ataxia telangiectasia-mutated and Rad3-related (ATR) kinase. The Journal of Biological Chemistry, 286, 28707–28714. https://doi.org/10.1074/jbc.M111.248914
Nam, E. A., & Cortez, D. (2011). ATR signalling: More than meeting at the fork. The Biochemical Journal, 436, 527–536. https://doi.org/10.1042/bj20102162
Navadgi-Patil, V. M., & Burgers, P. M. (2011). Cell-cycle-specific activators of the Mec1/ATR checkpoint kinase. Biochemical Society Transactions, 39, 600–605. https://doi.org/10.1042/bst0390600
Nayak, A., & Müller, S. (2014). SUMO-specific proteases/isopeptidases: SENPs and beyond. Genome Biology, 15, 422. https://doi.org/10.1186/s13059-014-0422-2
Oakley, G. G., et al. (2009). Physical interaction between replication protein A (RPA) and MRN: Involvement of RPA2 phosphorylation and the N-terminus of RPA1. Biochemistry, 48, 7473–7481. https://doi.org/10.1021/bi900694p
Ohashi, E., Takeishi, Y., Ueda, S., & Tsurimoto, T. (2014). Interaction between Rad9-Hus1-Rad1 and TopBP1 activates ATR-ATRIP and promotes TopBP1 recruitment to sites of UV-damage. DNA Repair, 21, 1–11. https://doi.org/10.1016/j.dnarep.2014.05.001
Peschiaroli, A., et al. (2006). SCFbetaTrCP-mediated degradation of Claspin regulates recovery from the DNA replication checkpoint response. Molecular Cell, 23, 319–329. https://doi.org/10.1016/j.molcel.2006.06.013
Post, S., et al. (2001). Phosphorylation of serines 635 and 645 of human Rad17 is cell cycle regulated and is required for G(1)/S checkpoint activation in response to DNA damage. Proceedings of the National Academy of Sciences of the United States of America, 98, 13102–13107. https://doi.org/10.1073/pnas.231364598
Primo, L. M. F., & Teixeira, L. K. (2019). DNA replication stress: Oncogenes in the spotlight. Genetics and Molecular Biology, 43, e20190138. https://doi.org/10.1590/1678-4685gmb-2019-0138
Reyes-Turcu, F. E., Ventii, K. H., & Wilkinson, K. D. (2009). Regulation and cellular roles of ubiquitin-specific deubiquitinating enzymes. Annual Review of Biochemistry, 78, 363–397. https://doi.org/10.1146/annurev.biochem.78.082307.091526
Rodríguez-Paredes, M., & Lyko, F. (2019). The importance of non-histone protein methylation in cancer therapy. Nature Reviews. Molecular Cell Biology, 20, 569–570. https://doi.org/10.1038/s41580-019-0147-x
Roos-Mattjus, P., et al. (2002). Genotoxin-induced Rad9-Hus1-Rad1 (9-1-1) chromatin association is an early checkpoint signaling event. The Journal of Biological Chemistry, 277, 43809–43812. https://doi.org/10.1074/jbc.M207272200
Roos-Mattjus, P., et al. (2003). Phosphorylation of human Rad9 is required for genotoxin-activated checkpoint signaling. The Journal of Biological Chemistry, 278, 24428–24437. https://doi.org/10.1074/jbc.M301544200
Rundle, S., Bradbury, A., Drew, Y., & Curtin, N. J. (2017). Targeting the ATR-CHK1 axis in cancer therapy. Cancers. https://doi.org/10.3390/cancers9050041
Sato, K., et al. (2012). A DNA-damage selective role for BRCA1 E3 ligase in claspin ubiquitylation, CHK1 activation, and DNA repair. Current Biology: CB, 22, 1659–1666. https://doi.org/10.1016/j.cub.2012.07.034
Shanmugam, I., et al. (2014). Ubiquitin-specific peptidase 20 regulates Rad17 stability, checkpoint kinase 1 phosphorylation and DNA repair by homologous recombination. The Journal of Biological Chemistry, 289, 22739–22748. https://doi.org/10.1074/jbc.M114.550459
Shiotani, B. & Zou, L. (2009). ATR signaling at a glance. Journal of Cell Science, 122, 301–304. https://doi.org/10.1242/jcs.035105
Shiotani, B., et al. (2013). Two distinct modes of ATR activation orchestrated by Rad17 and Nbs1. Cell Reports, 3, 1651–1662. https://doi.org/10.1016/j.celrep.2013.04.018
Singh, M., Burrows, A. C., Torres, A. E., Pal, D., Stromberg, B., Insinna, C., Dickson, A., Westlake, C. J., Summers, M. K. (2019). The deubiquitinating enzyme USP37 stabilizes CHK1 to promote the cellular response to replication stress. bioRxiv 652891.
Smith, J., Tho, L. M., Xu, N., & Gillespie, D. A. (2010). The ATM-Chk2 and ATR-Chk1 pathways in DNA damage signaling and cancer. Advances in Cancer Research, 108, 73–112. https://doi.org/10.1016/b978-0-12-380888-2.00003-0
Sørensen, C. S., & Syljuåsen, R. G. (2012). Safeguarding genome integrity: The checkpoint kinases ATR, CHK1 and WEE1 restrain CDK activity during normal DNA replication. Nucleic Acids Research, 40, 477–486. https://doi.org/10.1093/nar/gkr697
Sun, J., Shi, X., Mamun, M. A. A., & Gao, Y. (2020). The role of deubiquitinating enzymes in gastric cancer. Oncology Letters, 19, 30–44. https://doi.org/10.3892/ol.2019.11062
Sun, T., Liu, Z., & Yang, Q. (2020). The role of ubiquitination and deubiquitination in cancer metabolism. Molecular Cancer, 19, 146. https://doi.org/10.1186/s12943-020-01262-x
Surendran, S., Ononye, O. E., & Balakrishnan, L. (2016). Acetylation of replication protein A (RPA) improves its DNA binding property. The FASEB Journal.
Swatek, K. N., & Komander, D. (2016). Ubiquitin modifications. Cell Research, 26, 399–422. https://doi.org/10.1038/cr.2016.39
Ulrich, H. D., & Walden, H. (2010). Ubiquitin signalling in DNA replication and repair. Nature Reviews. Molecular Cell Biology, 11, 479–489. https://doi.org/10.1038/nrm2921
Walter, J., & Newport, J. (2000). Initiation of eukaryotic DNA replication: origin unwinding and sequential chromatin association of Cdc45, RPA, and DNA polymerase alpha. Molecular Cell, 5, 617–627. https://doi.org/10.1016/s1097-2765(00)80241-5
Wan, J., Liu, H., Chu, J., & Zhang, H. (2019). Functions and mechanisms of lysine crotonylation. Journal of Cellular and Molecular Medicine, 23, 7163–7169. https://doi.org/10.1111/jcmm.14650
Wang, R. H., et al. (2014). SIRT1 deacetylates TopBP1 and modulates intra-S-phase checkpoint and DNA replication origin firing. International Journal of Biological Sciences, 10, 1193–1202. https://doi.org/10.7150/ijbs.11066
Wang, X., et al. (2006). Rad17 phosphorylation is required for claspin recruitment and Chk1 activation in response to replication stress. Molecular Cell, 23, 331–341. https://doi.org/10.1016/j.molcel.2006.06.022
Wang, X., et al. (2017). 3.9 Å structure of the yeast Mec1-Ddc2 complex, a homolog of human ATR-ATRIP. Science (New York, N.Y.), 358, 1206–1209. https://doi.org/10.1126/science.aan8414
Wardlaw, C. P., Carr, A. M., & Oliver, A. W. (2014). TopBP1: A BRCT-scaffold protein functioning in multiple cellular pathways. DNA Repair, 22, 165–174. https://doi.org/10.1016/j.dnarep.2014.06.004
Wilhelm, T., Said, M., & Naim, V. (2020). DNA replication stress and chromosomal instability: Dangerous liaisons. Genes. https://doi.org/10.3390/genes11060642
Wilkinson, K. D. (2000). Ubiquitination and deubiquitination: Targeting of proteins for degradation by the proteasome. Seminars in Cell & Developmental Biology, 11, 141–148. https://doi.org/10.1006/scdb.2000.0164
Wu, C. S., et al. (2014). SUMOylation of ATRIP potentiates DNA damage signaling by boosting multiple protein interactions in the ATR pathway. Genes & Development, 28, 1472–1484. https://doi.org/10.1101/gad.238535.114
Wu, W., et al. (2018). HERC2 facilitates BLM and WRN helicase complex interaction with RPA to suppress G-Quadruplex DNA. Cancer Research, 78, 6371–6385. https://doi.org/10.1158/0008-5472.Can-18-1877
Xia, C., Tao, Y., Li, M., Che, T., & Qu, J. (2020). Protein acetylation and deacetylation: An important regulatory modification in gene transcription (Review). Experimental and Therapeutic Medicine, 20, 2923–2940. https://doi.org/10.3892/etm.2020.9073
Yamane, K., Wu, X., & Chen, J. (2002). A DNA damage-regulated BRCT-containing protein, TopBP1, is required for cell survival. Molecular and Cellular Biology, 22, 555–566. https://doi.org/10.1128/mcb.22.2.555-566.2002
Yang, C. C., Kato, H., Shindo, M., & Masai, H. (2019). Cdc7 activates replication checkpoint by phosphorylating the Chk1-binding domain of Claspin in human cells. eLife. https://doi.org/10.7554/eLife.50796
Yang, J., et al. (2004). ATM and ATR: Sensing DNA damage. World Journal of Gastroenterology, 10, 155–160. https://doi.org/10.3748/wjg.v10.i2.155
Yang, X. H., & Zou, L. (2006). Recruitment of ATR-ATRIP, Rad17, and 9-1-1 complexes to DNA damage. Methods in Enzymology, 409, 118–131. https://doi.org/10.1016/s0076-6879(05)09007-5
Yang, Y., et al. (2017). Protein SUMOylation modification and its associations with disease. Open Biology. https://doi.org/10.1098/rsob.170167
Yazinski, S. A., & Zou, L. (2016). Functions, regulation, and therapeutic implications of the ATR checkpoint pathway. Annual Review of Genetics, 50, 155–173. https://doi.org/10.1146/annurev-genet-121415-121658
Young, M. J., Hsu, K. C., Lin, T. E., Chang, W. C., & Hung, J. J. (2019). The role of ubiquitin-specific peptidases in cancer progression. Journal of Biomedical Science, 26, 42. https://doi.org/10.1186/s12929-019-0522-0
Yu, H., et al. (2020). Global crotonylome reveals CDYL-regulated RPA1 crotonylation in homologous recombination-mediated DNA repair. Sci Adv. https://doi.org/10.1126/sciadv.aay4697
Yu, X., et al. (2020). Ubiquitination of the DNA-damage checkpoint kinase CHK1 by TRAF4 is required for CHK1 activation. Journal of Hematology & Oncology, 13, 40. https://doi.org/10.1186/s13045-020-00869-3
Yu, X., Chini, C. C., He, M., Mer, G., & Chen, J. (2003). The BRCT domain is a phospho-protein binding domain. Science (New York, N.Y.), 302, 639–642. https://doi.org/10.1126/science.1088753
Yuan, J., et al. (2014). HERC2-USP20 axis regulates DNA damage checkpoint through Claspin. Nucleic Acids Research, 42, 13110–13121. https://doi.org/10.1093/nar/gku1034
Zhang, D., Zaugg, K., Mak, T. W., & Elledge, S. J. (2006). A role for the deubiquitinating enzyme USP28 in control of the DNA-damage response. Cell, 126, 529–542. https://doi.org/10.1016/j.cell.2006.06.039
Zhang, H., et al. (2016). ATRIP deacetylation by SIRT2 drives ATR checkpoint activation by promoting binding to RPA-ssDNA. Cell Reports, 14, 1435–1447. https://doi.org/10.1016/j.celrep.2016.01.018
Zhang, L., et al. (2010). Proteolysis of Rad17 by Cdh1/APC regulates checkpoint termination and recovery from genotoxic stress. The EMBO Journal, 29, 1726–1737. https://doi.org/10.1038/emboj.2010.55
Zhao, M., et al. (2017). PCAF/GCN5-Mediated Acetylation of RPA1 Promotes Nucleotide Excision Repair. Cell reports, 20, 1997–2009. https://doi.org/10.1016/j.celrep.2017.08.015
Zheng, N., Wang, Z., & Wei, W. (2016). Ubiquitination-mediated degradation of cell cycle-related proteins by F-box proteins. The International Journal of Biochemistry & Cell Biology, 73, 99–110. https://doi.org/10.1016/j.biocel.2016.02.005
Zhou, L., et al. (2020). SUMOylation stabilizes hSSB1 and enhances the recruitment of NBS1 to DNA damage sites. Signal Transduction and Targeted Therapy, 5, 80. https://doi.org/10.1038/s41392-020-0172-4
Zhu, M., Zhao, H., Liao, J., & Xu, X. (2014). HERC2/USP20 coordinates CHK1 activation by modulating CLASPIN stability. Nucleic Acids Research, 42, 13074–13081. https://doi.org/10.1093/nar/gku978
Zou, L., & Elledge, S. J. (2003). Sensing DNA damage through ATRIP recognition of RPA-ssDNA complexes. Science (New York, N.Y.), 300, 1542–1548. https://doi.org/10.1126/science.1083430
Acknowledgements
We gratefully acknowledge funding from National Natural Science Foundation(32070713 to J.Y., 82002985 to Y.P.C., 32090032 to J.Y.), Shanghai Pujiang program(2020PJD070 to Y.P.C.) and China Postdoctoral Science Foundation (2020M681384 to Y.P.C.).
Author information
Affiliations
Key Laboratory of Arrhythmias of the Ministry of Education of China, Research Center for Translational Medicine, East Hospital, School of Life Sciences and Technology, Shanghai, 200120, China
Yuping Chen
Key Laboratory of Arrhythmias of the Ministry of Education of China, Research Center for Translational Medicine, East Hospital, Tongji University School of Medicine, Shanghai, 200120, China
Yuping Chen & Jian Yuan
Department of Biochemistry and Molecular Biology, Tongji University School of Medicine, Shanghai, 200120, China
Yuping Chen & Jian Yuan
Corresponding author
Correspondence to Jian Yuan.
Ethics declarations
Conflict of interest
The authors declare that they have no competing interests.
Rights and permissions
About this article
Cite this article
Chen, Y., Yuan, J. The post translational modification of key regulators of ATR signaling in DNA replication. GENOME INSTAB. DIS. 2, 92–101 (2021). https://doi.org/10.1007/s42764-021-00036-z
Received09 February 2021
Revised03 March 2021
Accepted08 March 2021
Published21 April 2021
Issue DateApril 2021
Share this article
Anyone you share the following link with will be able to read this content:
Get shareable linkKeywords
Post-translational modification
ATR pathway
DNA replication
DNA replication stress
用户登录
还没有账号?
立即注册