Microproteins: from behind the scenes to the spotlight
Review Article
Genome Instability & Disease , 2 225–239 (2021)
Abstract
Microproteins, less than 200 amino acids, have been usually regarded as non-functional for a long time. We do not recognize this “dark matter” in the proteome until developing new bioinformatic, biochemical and proteomic techniques. The fast-growing number of the identified microproteins and their emerging roles in various biological processes begin to attract more and more attention. Here, we summarize the recent progress in this field mainly from four aspects: (1) brief history and various definitions of microproteins; (2) three main strategies to identify microproteins; (3) multiple regulatory mechanisms of microproteins; (4) biological functions of microproteins, with a particular focus on genome stability maintenance and their relevance to human diseases. Finally, we discuss some open questions and therapeutic opportunities hiding in these small newcomers.
Introduction
In this review, we define “microproteins” as functional proteins from 2 amino acids (aa) and up to 200 aa, which are usually encoded by small open reading frames (sORFs/smORFs) (Basrai et al., 1997; Magnani et al., 2014; Yang et al., 2011). Nowadays, researchers in this field agree that microproteins are small in size, numerous in variety with underestimated functions thus far due to several reasons. First, microproteins are routinely discarded during the automatic gene annotation process in the past because smORFs less than 100 codons are considered untranslatable and “meaningless” in the eukaryotic genomes (Basrai et al., 1997; Chugunova et al., 2018; Frith et al., 2006). Second, eukaryotic mRNA is often mono-cistronic that encodes a single protein, while the bacterial transcript is poly-cistronic that encodes several proteins. It was well-acknowledged in eukaryotic cells that canonical proteins are translated from mRNA; thus, alternative transcription and translation are overlooked.
The pioneering systematical studies on microproteins started from yeast in 1997 (Basrai et al., 1997). Shortly after that, the Boeke group reported functional analysis of smORFs in the yeast genome and identified several microproteins that respond to different stress (Kastenmayer et al., 2006). Then, Savard et al. (2006) found that mlpt mRNA is poly-cistronic and encodes four small peptides. Three of them shared conserved motif, and depletion of mlpt impaired the embryo segmentation in Tribolium. Subsequently, two groups independently reported that the homology gene of mlpt in Drosophila, tal/pri, has similar biological functions (Galindo et al., 2007; Kondo et al., 2007). These pioneer studies kick off a microprotein gold rush, especially in higher eukaryotes.
With the development of techniques including bioinformatic methods, mass spectrometry and newly developed ribosome profiling (Andrews & Rothnagel, 2014; Couso & Patraquim, 2017; Ingolia et al., 2009; Makarewich & Olson, 2017; Orr et al., 2020; Peeters & Menschaert, 2020; Plaza et al., 2017; Pueyo et al., 2016; Schlesinger & Elsasser, 2021; Sousa & Farkas, 2018; Xiao et al., 2018), thousands of translated smORFs or alternative-ORFs (alt-ORFs) in the genomes have been identified thus far. Surprisingly, microproteins are encoded by mRNAs as well as rRNA and other kinds of RNA that were previously considered noncoding (Non-coding RNA, ncRNA), including alternative transcripts and non-AUG start codon initiation transcripts, uORF (upstream ORF) located in the 5’ UTR of mRNA and circular RNA (circRNA) (Table 1).
Table 1 Names and definitions of microproteinsFull size tableAs an emerging and rapidly evolving field, there are many different definitions, classifications, and nomenclatures of “smORF-coded proteins” (Table 1). For example, according to (1) their small size, from 2 to 200 aa, “microprotein” (Makarewich, 2020; Rathore et al., 2018), “small protein” (Basrai et al., 1997; Oyama et al., 2004; Storz et al., 2014), “micropeptide” (Chen et al., 2021; Makarewich & Olson, 2017; Vitorino et al., 2021), “miniproteins” (Crook et al., 2020); (2) their original sources, “SEP” (smORF-encoded polypeptide) (Pueyo et al., 2016; Saghatelian & Couso, 2015; Yin et al., 2019) or “MDPs” (mitochondria-derived peptides) (Kim et al., 2017); (3) their “microRNAs-like” functions, that they bind and inhibit targeted proteins as negative regulators at the protein level, such as “microProtein, miP” (Bhati et al., 2018; Staudt & Wenkel, 2011), “inhibitor of DNA binding, Id proteins” (Roschger & Cabrele, 2017); (4) the unique structure or function, “cyclotide” was named after its head-to-tail cyclized backbone (Camarero, 2017; Craik & Du, 2017); (5) personalized naming like “CASIMO1”, “CYREN”, “DYNLL1” (Arnoult et al., 2017; He et al., 2018; Polycarpou-Schwarz et al., 2018).
Identification of microproteins
Bioinformatic prediction
Bioinformatic prediction is often based on characteristics of the coding regions distinct from randomly generated short fragments in the genome. The coding regions are more likely conserved than random parts, which can be evaluated by three parameters. First, the cross-species comparison is a useful strategy for gene prediction. Metrics like the Ka/Ks ratio test were developed to evaluate the conservation of the coding sequence (Hurst, 2002). The Ka/Ks ratio test is used based on the fact that synonymous substitutions (Ks) occur much more frequently than nonsynonymous ones (Ka) in most of the coding regions. If the protein sequence is conserved between species, the Ka/Ks ratio is usually less than 1. Second, proteins often contain conserved domain/motif(s). Third, to analyze the sequence content and characteristics features, such as Kozak sequence, codon usage bias, nucleotide composition and so on (Gelfand, 1995). Thus, stringent selection can be implemented according to these sequence signatures. Based on the protein characters mentioned above, several smORF prediction tools have been successfully developed to improve the sensitivity and accuracy of smORF prediction, such as sORF Finder (Hanada et al., 2010), FSPP (Li et al., 2018), PhyloCSF (Lin et al., 2011) and CRITICA (Frith et al., 2006). However, whether such in silico predicted smORFs could be translated into bioactive microproteins in vivo needs validation by wet-lab experiments.
Mass spectrometry (MS)
In principle, MS is a widely used and practical proteomic approach. However, the traditional MS has two major disadvantages in microprotein identification. First, MS relies on mapping the MS spectra of digested peptides to the reference database generated by the theoretical spectra of annotated proteins (Zhang et al., 2013). Most of the translated microproteins are undetected just because they are unannotated. Second, the properties of microproteins, e.g., low abundance, low molecular weight (Andrews & Rothnagel, 2014) and instability, make them hard to be detected by MS (Slavoff et al., 2013). Third, sample preparation also impacts the MS results. Insufficient separation of analytes and inefficient enrichment of target proteins always comprise the detection of microproteins.
To overcome these challenges, researchers have adopted multiple strategies to improve the traditional MS. One advancement is the application of electrostatic repulsion hydrophilic interaction chromatography (ERLIC); it helps to separate the peptide mixture into a single component better and enrich the target peptides (Chugunova et al., 2018; Hao et al., 2011). The Slavoff group utilized the MWCO (Molecular weight cut-off) + ERLIC + LC–MS/MS workflow, that combined with a custom proteomic database based on the six-frame translation information generated from the RNA-seq data of K562 cells, and have successfully identified 195 novel microproteins in K562 cells (Ma et al., 2014). To find the most effective workflow, they also optimize the isolation and enrichment procedures before MS detection. By comparing four different extraction procedures and three enrichment steps, Slavoff et al. recommend combining acid precipitation and reverse-phase (C8) cartridges to enrich microproteins (Ma et al., 2016). These improvements lead to better identification of microproteins in human cells.
Yang et al. (2011) developed a three-step strategy that combines computational, transcriptional, and translational methods to identify protein-coding smORF in the Populus genome. They first utilized expressed sequence tags (EST) to construct a custom database, then filtered all candidates by three computational programs based on the coding potential, cross-species conservation, and protein sequence clustering. After a validation step by mass spectrometry, they finally designated 611 high confidence protein-coding smORFs, 56 of which are new to the Populus genome.
Ribosome profiling (Ribo-seq)
Ribo-seq is a powerful and emerging biochemical technology for quantifying global translation conditions in cells. It is developed by Ingolia et al. (2009), based on the fact that ribosomes often bind actively translated mRNAs. Ribo-seq utilizes next-generation sequencing (NGS) to analyze the 22–35 nt mRNA protected from nuclease digestion by the ribosome. Then, the ribosome protected fragment (RPF) is mapped back to the genomic loci with density information reflecting the translation efficiency (Aspden et al., 2014).
Ribo-seq has been used to identify a large number of smORFs in various species like yeast (Ingolia et al., 2009), fruit fly (Aspden et al., 2014), zebrafish (Bazzini et al., 2014), mouse (Ingolia et al., 2011) and human (Gonzalez et al., 2014; Raj et al., 2016). Although ribosome profiling has several advantages over other methods in smORF detection, it still has some limitations. For example, RNA binding proteins and the stalled or inactive ribosomes can also bind mRNA that may result in false positives. Therefore, poly-Ribo-seq was developed to detect smORFs specifically. The actively translating canonical CDS will be covered by multiple ribosomes called polysomes, while smORF is very short in length, so it usually accommodates no more than six ribosomes. Ultracentrifugation was used to separate and enrich the small polysomes (2–6) from large polysomes (Aspden et al., 2014). Aspden et al. applied this method in Drosophila S2 cells and identified 228 translated smORFs, compared to only 60 small peptides identified by mass spectrometry. Other derivative approaches such as TARP (Translating ribosome affinity purification)-seq (Heiman et al., 2014), RiboLace (Clamer et al., 2018) have been developed for cell-type-specific translational analysis. Through optimizing sample preparation and combining multiple strategies, we have witnessed a fast-growing number of identified microproteins in the last decade.
Biological function of microproteins
Although the functional study of microproteins is still in its infancy, microproteins have been implicated in a wide range of biological processes including embryonic development (Kondo et al., 2007, 2010), muscle formation and regeneration (Anderson et al., 2015; Magny et al., 2013; Matsumoto et al., 2017; Nelson et al., 2016), cellular stress response (Chu et al., 2019; Khitun et al., 2019; Starck et al., 2016), RNA regulation (D'Lima et al., 2017) and DNA repair (Arnoult et al., 2017; Slavoff et al., 2014). This section will elaborate on the regulation of protein function by microproteins at multiple levels, including transcription, translation and post-translational modifications, with a particular focus on their contribution to genome stability maintenance processes including DNA replication and DNA double-strand break (DSB) repair.
Microproteins function at the transcriptional level
Microproteins can regulate gene expression at the transcriptional level by forming inactive complexes or competing for DNA/RNA binding (Staudt & Wenkel, 2011).
Id proteins
One of the most representative examples is Id proteins that inhibit the DNA binding activity of the basic Helix-Loop-Helix (bHLH) transcription factors (TFs). The bHLH TFs contain a basic region that binds to DNA and an HLH region that forms a homodimer or heterodimer with another bHLH TF. The monomeric bHLH TF has no transcription activity until forming homodimers or heterodimers. Id proteins contain only an HLH domain but lack the basic region. Therefore, Id proteins can form a non-functional dimer with a bHLH TF and result in a dominant-negative effect (Ruzinova & Benezra, 2003) (Fig. 1a). For example, Id2 interacts with the bHLH TFs CLOCK and BMAL to prevent these proteins from forming a functional dimer that binds to the E-box element responsible for downstream transcription of PER1 and CRY in the core mammalian circadian clock (Duffield et al., 2009).
Fig. 1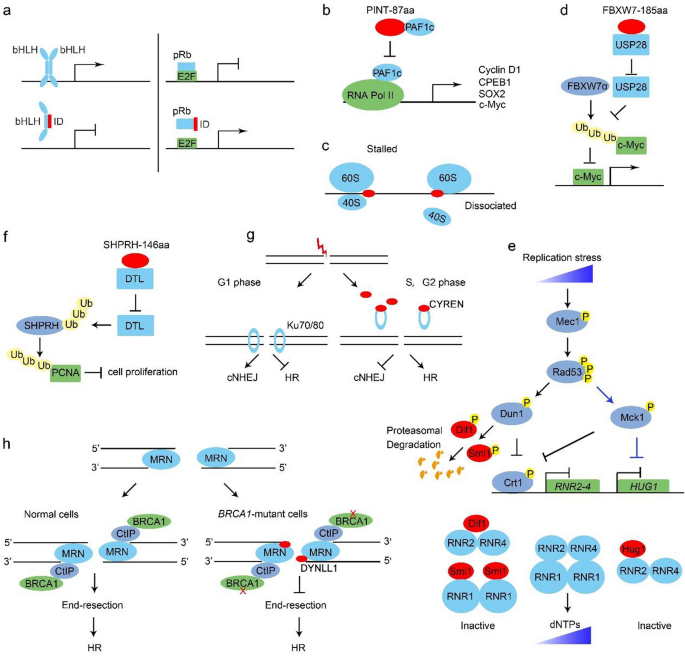
The biological functions of microproteins. a Id proteins form a non-functional dimer with a bHLH TF and result in transcription repression (left). Id2 interacts with pRb and releases E2F for transcription (right). b PINT-87aa directly binds the PAF1c and disrupts the RNA Pol II-PAF1c complex formation. c Microproteins cause ribosome stalling or dissociation. d FBXW7-185aa binds USP28 to antagonize its de-ubiquitination of c-Myc and free FBWX7α to targeting c-Myc for ubiquitination degradation. e Hug1, Sml1 and Dif1 regulate the dNTP level upon replicative stress. Under low or intermediate replication stress, both Dun1 and Mck1 can phosphorylate the transcriptional repressor Crt1 and thereby de-repress the RNR and HUG1 gene transcription. Dun1 also triggers a phosphorylation-dependent degradation of RNR inhibitors Sml1 and Dif1. In the presence of more severe stress, Mck1 inhibits the HUG1 transcription to boost dNTP levels further. f SHPRH-146aa protects full-length SHPRH from degradation mediated by E3 ligase DTL and promotes SHPRH-mediated ubiquitination and degradation of PCNA. g The recruitment of Ku70/80 to the DSB sites is diminished by CYREN association, which explains why cNHEJ is inhibited in S and G2 phases. h DYNLL1 interacts with Mre11 to impair the DNA end resection activities in BRCA1-deficient cancer cells
Full size imageBesides bHLH proteins, Id proteins also bind to and inhibit other transcription factors. Several studies have reported that Id2 interacts with the retinoblastoma protein (pRb), a transcriptional repressor controlling the genes required for G1/S transition. These indicate that Id2 can promote cell cycle progression and thereby antagonize the tumor suppressor function of pRb (Iavarone et al., 1994). This finding exemplifies that some microproteins can be exploited by cancer cells to avoiding growth suppressors (Fig. 1a).
PINT-87aa
Following the pioneering work in Id proteins, other microproteins like PINT-87aa have been found to regulate transcription through distinct mechanisms. The long intergenic non-protein-coding RNA p53-induced transcript (LINC-PINT) was found to have a circular form that encodes an 87-aa microprotein, PINT-87aa. PINT-87aa can directly bind the polymerase-associated factor complex (PAF1c) and prevent the RNA PolII-PAF1c complex formation (Fig. 1b), which is required for the transcription elongation of many oncogenes including Cyclin D1, c-Myc and Sox2. Consistently, PINT-87aa depletion increases tumor burden in vivo, suggesting that the microprotein PINT-87aa might be a candidate tumor suppressor (Zhang et al., 2018b).
Microproteins function at the translational level
In addition to the transcriptional control, accumulating evidence supports a prominent contribution of translational regulation in gene expression especially in eukaryotes. Microproteins encoded by uORFs have been found to pose a critical role in the mRNA translation process (Zhang et al., 2021). In the human genome, about 35% of genes contain uORFs. Recent findings suggest that microproteins translated from the uORFs often interact and interfere with ribosomes, leading to ribosome dissociation (start codon skipping) or stalling (Orr et al., 2020) (Fig. 1c). For example, the 5’ UTR of EPHX1 (Epoxide hydrolase 1) transcripts E1-b’ encode two microproteins of 26 and 17 aa in length, respectively. These two microproteins, in cis and in trans configurations, inhibit the EPHX1 translation (Nguyen et al., 2013). There is also a report that 5’ UTR-encoded microproteins (e.g. uORF of the McKusick-Kaufman syndromegene, uMKKS protein) may be directly involved in cellular processes, rather than translational regulation, albeit with unknown mechanisms (Akimoto et al., 2013).
Microproteins function at the post-translational level
The degradation and half-life of c-Myc proto-oncoprotein are strictly controlled by ubiquitination ligase FBXW7 (F-box and WD repeat domain containing 7) and de-ubiquitin ligase USP28. CircRNA FBXW7 encodes a microprotein called FBXW7-185aa, which has a higher affinity for USP28 and inhibits the interaction between USP28 and FBXW7α, and indirectly increases FBXW7-mediated ubiquitination of c-Myc (Fig. 1d). By this means, FBXW7-185aa limits the c-Myc protein level and expression of its downstream cell proliferation genes (Yang et al., 2018). Together with the abovementioned PINT-87aa, FBXW7-185aa exemplifies that microproteins can inhibit oncogene overexpression and proliferative signals required by cancer cells.
Microproteins function in DNA replication
Hug1, Sml1 and Dif1
Several microproteins have been found to fine-tune the cellular levels of deoxynucleotide triphosphates (dNTPs), crucial for the high fidelity of DNA replication and genomic stability maintenance. The rate-limiting step in the de novo dNTP synthesis is catalyzing the reduction of nucleotide diphosphates (NDPs) to deoxynucleotide diphosphates (dNDPs) by the ribonucleotide reductase (RNR). Yeast RNR is a hetero-tetrameric complex comprising two large subunits (Rnr1 or Rnr3) and two small subunits (Rnr2 and Rnr4). Rnr3 is usually repressed by Crt1 and only induced by replication stress or DNA damage.
Several microproteins regulate RNR at the protein level (Fig. 1e). Hug1, a 68 aa microprotein, binds the small subunit Rnr2 and inhibits the RNR activity (Meurisse et al., 2014). Recently, our group showed that the basal Hug1 protein level is very low under normal conditions. When cells encounter moderated replication stress, the Mec1-Rad53 checkpoint pathway phosphorylates Dun1 and the GSK-3 family kinase, Mck1. Both Mck1 and Dun1 phosphorylate Crt1 to release its suppression of RNR2-4 and HUG1 transcription. The RNR inhibitory role of Hug1 may function as a rheostat to prevent excessive dNTP production, which increases genome instability as well. When cells suffer the more severe or persistent stress, Mck1 can inhibit the HUG1 transcription in a Crt1-independent manner to further augment the RNR activity and dNTP levels (Li et al., 2019).
Besides Hug1, two paralogous microproteins, Sml1 and Dif1, regulate RNR as well. Sml1 hijacks Rnr1 in the cytoplasm and prevents the formation of RNR holoenzyme (Chabes et al., 1999; Zhao et al., 2001). Dif1 bears a HUG motif similar to Hug1. It can bind the Rnr2/Rnr4 heterodimer and mediate its nucleus import, preventing its association with large subunits to form a functional RNR complex in the cytoplasm (Lee et al., 2008; Wu & Huang, 2008).
In summary, these microproteins regulate the RNR activity and cellular dNTP levels in a tunable and scalable manner and thus make a significant contribution to maintaining genome integrity under both unperturbed and perturbed conditions.
SHPRH-146aa
Besides the building blocks for DNA replication, some key replisome components are regulated by microproteins as well. PCNA (Proliferating cell nuclear antigen) plays indispensable roles as the clamp of DNA polymerases and a hub for nearly all DNA-related activities. Its protein level is regulated by SHPRH (SNF2 histone linker PHD RING helicase) ubiquitin ligase E3. Recently, Zhang et al. identified a circular RNA (circ-SHPRH) transcript in the SHPRH gene locus encoding a 146 aa microprotein, SHPRH-146aa. SHPRH-146aa interacts with the E3 ligase denticleless (DTL) and inhibits DTL-mediated ubiquitin–proteasome degradation of SHPRH (Fig. 1f). By this mechanism, SHPRH-146aa negatively regulates the PCNA protein level. SHPRH-146aa is downregulated in glioblastoma. Overexpression of SHPRH-146aa in glioblastoma cells inhibits its viability and tumorigenicity (Zhang et al., 2018a). These findings indicate that microproteins like SHPRH-146aa and PINT-87aa can function as tumor suppressors and therefore represent potential therapeutic implications.
Microproteins function in the DNA double-strand break (DSB) repair
DSBs, the most severe type of DNA damage, are mainly repaired by the canonical non-homologous end joining (cNHEJ) and homologous recombination (HR) repair pathways. To date, several microproteins have been reported as critical regulators of the DSB repair pathway choices, such as CYREN1/2/3, DYNLL1 and AltMRVI1.
CYREN-1/2/3
Three isoforms of CYREN (cell cycle regulator of NHEJ)/MRI (modulator of retroviral infection) are encoded by C7orf49 in mammals. CYREN/MRI-2 was first shown to stimulate cNHEJ through interaction with the key cNHEJ determinant factor Ku70/80 (Slavoff et al., 2014). Karlseder and colleagues later found that CYREN-1 and CYREN-2 bind Ku70/80 only in S and G2 phases. Instead, the association of CYREN diminishes the recruitment of Ku70/80 to the DSB sites, which creates an environment favorable for error-free homologous recombination between sister chromatids while inhibiting cNHEJ (Fig. 1g). The deletion of CYREN enables cNHEJ to occur, promoting chromosome fusion at telomeres, specifically during S and G2 phases (Arnoult et al., 2017).
DYNLL1
In contrast to the HR-promoting role of CYREN, DYNLL1 (Dynein light chain 1 protein) directly inhibits the HR pathway during DSB repair. DYNLL1 is an 8 kDa light chain protein of the cytoplasmic dynein protein complex. The most well-acknowledged function of DYNLL1 is to act as a cargo adaptor of the motor complexes like dynein and myosin (Rapali et al., 2011).
In a genome-scale screen using CRISPR Cas9-based knockout library, Chowdhury and his colleague uncovered that loss of DYNLL1 shows strong resistance to PARPi and platinum drugs in BRCA1-deficient cancer cells. As BRCA1 is vital in the HR repair pathway, this result implicates that DYNLL1 plays a negative role in regulating the HR pathway. In molecular detail, they demonstrate that DYNLL1 directly interacts with MRE11 to inhibit its endonuclease activities, which is required for DNA end resection and subsequent HR (He et al., 2018). DYNLL1 functions as a potent inhibitor of DNA end resection when HR is impaired in BRCA1-deficient cancer cells. These findings suggest that DYNLL1 is responsible for resistance to PARPi or platinum chemotherapy and might be a possible candidate target for synthetic lethality-based cancer therapy (Fig. 1h).
Another microprotein, AltMRVI1 encoded by the 3’ UTR of the MRVI1 mRNA, was reported to interact with BRCA1 (Vanderperre et al., 2013). However, the exact role of AltMRVI1 awaits to be investigated.
The studies mentioned above establish the critical contribution of microproteins to avoiding genome instability and uncontrolled cell proliferation, which are hallmarks of many human diseases like cancer. Indeed, the abnormal expression of microproteins has been found to be associated with calcium homeostasis in muscles, obesity, diabetes, tumors and neurodegenerative diseases (Table 2). Interested readers can refer to the recent excellent reviews on this topic (Chen et al., 2021; Merino-Valverde et al., 2020; Wang et al., 2019; Wu et al., 2020a; Zhou et al., 2021).
Table 2 Microproteins in human diseasesFull size tableConclusions and future perspectives
As the “dark matter” in the proteome, microproteins are not recognized till the recent development of cutting-edge techniques (Fig. 2a). In this minireview, we have briefly summarized the recent technique improvements and research progresses in identifying and characterizing microproteins, with a particular focus on their function in maintaining genome integrity (Fig. 2b).
Fig. 2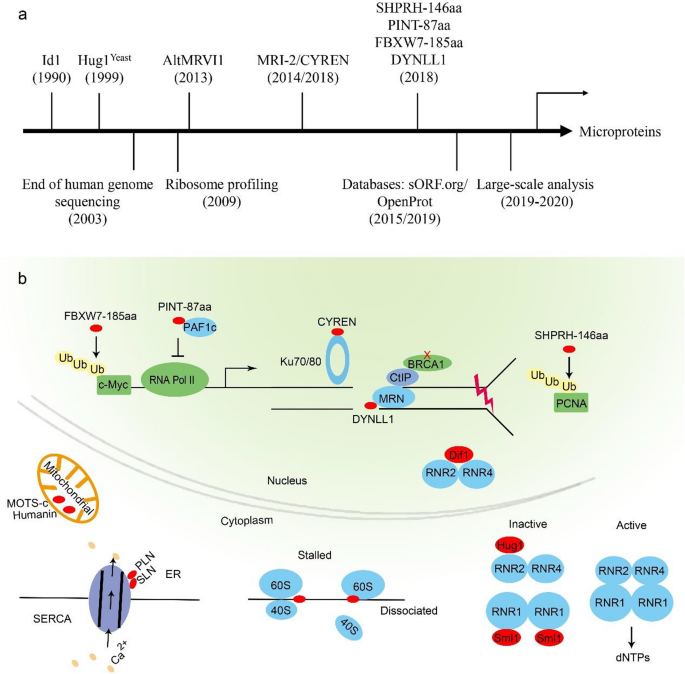
A brief depiction of the microprotein research (a) and biological functions discussed in this review (b)
Full size imageAs an emergent field, many aspects of microproteins wait to be addressed in the future.
1.
Standard nomenclature and guidelines are necessary for future research on microproteins with different origins in different organisms.
2.
Compare to an increasing number of microproteins that have been identified, our knowledge of their functions and molecular mechanisms is still very limited. For example, the Hieter group identified 302 unannotated ORFs (NORFs) in Saccharomyces cerevisiae in 1999 (Basrai et al., 1999). Among them, only the function of NORF5/HUG1 has been studied. The true challenge in this field is a focus shift from identification to functional studies.
3.
Microproteins usually lack a conserved domain and more likely contain flexible structures, making their functions more challenging to address. Moreover, epitope tagging, as one of the most common methods for protein functional analysis, will more likely interfere with the physiological properties of microproteins than that of regular proteins.
4.
Besides their physiological function, the pathological significance of microproteins will be of great interest to explore. For instance, the understanding of disease-specific microproteome, dysregulation and mutation landscape of microproteins will hold promise for novel markers, drugs and therapeutic targets.
5.
Microproteins are very small in size, and many of them are cell membrane permeable. This unique character will endow them with unparalleled advantages in drug discovery or delivery.
Abbreviations
aa:
Amino acids
alt-ORFs:
Alternative-ORFs
bHLH:
Basic helix-loop-helix
CASIMO1:
Cancer-associated small integral membrane open reading frame 1
circRNA:
Circular RNA
cNHEJ:
Canonical non-homologous end joining
CYREN:
Cell cycle regulator of NHEJ
dNDPs:
Deoxynucleotide diphosphates
dNTPs:
Deoxynucleotide triphosphates
DTL:
Denticleless
DSB:
DNA double-strand break
DYNLL1:
Dynein light chain LC8-type 1
EPHX1:
Epoxide hydrolase 1
ER:
Endoplasmic reticulum
ERLIC:
Electrostatic repulsion hydrophilic interaction chromatography
EST:
Expressed sequence tags
FBXW7:
F-box and WD repeat domain containing 7
HR:
Homologous recombination
Id:
Inhibitor of DNA binding and/or differentiation
LINC-PINT:
The long intergenic non-protein-coding RNA p53-induced transcript
MDPs:
Mitochondria-derived peptides
miPs:
MicroProteins
MRI:
Modulator of retroviral infection
MS:
Mass spectrometry
MWCO:
Molecular weight cut-off
ncRNA:
Non-coding RNA
NDPs:
Nucleotide diphosphates
NGS:
Next-generation sequencing
NORFs:
Nonannotated ORFs
NOPs:
3' Neo open reading frame peptides
PAF1c:
Polymerase-associated factor complex
PCNA:
Proliferating cell nuclear antigen
pRb:
The retinoblastoma protein
RNR:
Ribonucleotide reductase
RPF:
Ribosome protected fragment
SEP:
smORF-encoded polypeptide
SHPRH:
SNF2 histone linker PHD RING helicase
sORFs/smORFs:
Small open reading frames
SR:
Sarcoplasmic reticulum
TARP:
Translating ribosome affinity purification
TFs:
Transcription factors
uORF:
Upstream ORF
uMKKS:
uORF of the McKusick-Kaufman syndrome gene
References
Akimoto, C., Sakashita, E., Kasashima, K., Kuroiwa, K., Tominaga, K., Hamamoto, T., & Endo, H. (2013). Translational repression of the McKusick-Kaufman syndrome transcript by unique upstream open reading frames encoding mitochondrial proteins with alternative polyadenylation sites. Biochimica Et Biophysica Acta, 1830, 2728–2738. https://doi.org/10.1016/j.bbagen.2012.12.010
Anderson, D. M., Anderson, K. M., Chang, C. L., Makarewich, C. A., Nelson, B. R., McAnally, J. R., Kasaragod, P., Shelton, J. M., Liou, J., Bassel-Duby, R., & Olson, E. N. (2015). A micropeptide encoded by a putative long noncoding RNA regulates muscle performance. Cell, 160, 595–606. https://doi.org/10.1016/j.cell.2015.01.009
Anderson, D. M., Makarewich, C. A., Anderson, K. M., Shelton, J. M., Bezprozvannaya, S., Bassel-Duby, R., & Olson, E. N. (2016). Widespread control of calcium signaling by a family of SERCA-inhibiting micropeptides. Science Signaling, 9, ra119. https://doi.org/10.1126/scisignal.aaj1460
Andrews, S. J., & Rothnagel, J. A. (2014). Emerging evidence for functional peptides encoded by short open reading frames. Nature Reviews Genetics, 15, 193–204. https://doi.org/10.1038/nrg3520
Arnoult, N., Correia, A., Ma, J., Merlo, A., Garcia-Gomez, S., Maric, M., Tognetti, M., Benner, C. W., Boulton, S. J., Saghatelian, A., & Karlseder, J. (2017). Regulation of DNA repair pathway choice in S and G2 phases by the NHEJ inhibitor CYREN. Nature, 549, 548–552. https://doi.org/10.1038/nature24023
Aspden, J. L., Eyre-Walker, Y. C., Phillips, R. J., Amin, U., Mumtaz, M. A., Brocard, M., & Couso, J. P. (2014). Extensive translation of small open reading frames revealed by poly-ribo-seq. eLife, 3, e03528. https://doi.org/10.7554/eLife.03528
Basrai, M. A., Hieter, P., & Boeke, J. D. (1997). Small open reading frames: beautiful needles in the haystack. Genome Research, 7, 768–771. https://doi.org/10.1101/gr.7.8.768
Basrai, M. A., Velculescu, V. E., Kinzler, K. W., & Hieter, P. (1999). NORF5/HUG1 is a component of the MEC1-mediated checkpoint response to DNA damage and replication arrest in Saccharomyces cerevisiae. Molecular and Cellular Biology, 19, 7041–7049. https://doi.org/10.1128/mcb.19.10.7041
Bazzini, A. A., Johnstone, T. G., Christiano, R., Mackowiak, S. D., Obermayer, B., Fleming, E. S., Vejnar, C. E., Lee, M. T., Rajewsky, N., Walther, T. C., & Giraldez, A. J. (2014). Identification of small ORFs in vertebrates using ribosome footprinting and evolutionary conservation. The EMBO Journal, 33, 981–993. https://doi.org/10.1002/embj.201488411
Bhati, K. K., Blaakmeer, A., Paredes, E. B., Dolde, U., Eguen, T., Hong, S. Y., Rodrigues, V., Straub, D., Sun, B., & Wenkel, S. (2018). Approaches to identify and characterize microProteins and their potential uses in biotechnology. Cellular and Molecular Life Sciences, 75, 2529–2536. https://doi.org/10.1007/s00018-018-2818-8
Bi, P., Ramirez-Martinez, A., Li, H., Cannavino, J., McAnally, J. R., Shelton, J. M., Sanchez-Ortiz, E., Bassel-Duby, R., & Olson, E. N. (2017). Control of muscle formation by the fusogenic micropeptide myomixer. Science, 356, 323–327. https://doi.org/10.1126/science.aam9361
Brunet, M. A., Leblanc, S., & Roucou, X. (2020). Reconsidering proteomic diversity with functional investigation of small ORFs and alternative ORFs. Experimental Cell Research, 393, 112057. https://doi.org/10.1016/j.yexcr.2020.112057
Cabrera-Quio, L. E., Herberg, S., & Pauli, A. (2016). Decoding sORF translation - from small proteins to gene regulation. RNA Biology, 13, 1051–1059. https://doi.org/10.1080/15476286.2016.1218589
Camarero, J. A. (2017). Cyclotides, a versatile ultrastable micro-protein scaffold for biotechnological applications. Bioorganic and Medicinal Chemistry Letters, 27, 5089–5099. https://doi.org/10.1016/j.bmcl.2017.10.051
Cao, X., Khitun, A., Luo, Y., Na, Z., Phoodokmai, T., Sappakhaw, K., Olatunji, E., Uttamapinant, C., & Slavoff, S. A. (2021). Alt-RPL36 downregulates the PI3K-AKT-mTOR signaling pathway by interacting with TMEM24. Nature Communications, 12, 508. https://doi.org/10.1038/s41467-020-20841-6
Cardon, T., Fournier, I., & Salzet, M. (2021). Shedding light on the ghost proteome. Trends in Biochemical Sciences, 46, 239–250. https://doi.org/10.1016/j.tibs.2020.10.003
Chabes, A., Domkin, V., & Thelander, L. (1999). Yeast Sml1, a protein inhibitor of ribonucleotide reductase. The Journal of Biological Chemistry, 274, 36679–36683. https://doi.org/10.1074/jbc.274.51.36679
Chen, Y., Ho, L., & Tergaonkar, V. (2021). sORF-Encoded MicroPeptides: New players in inflammation, metabolism, and precision medicine. Cancer Letters, 500, 263–270. https://doi.org/10.1016/j.canlet.2020.10.038
Chu, Q., Martinez, T. F., Novak, S. W., Donaldson, C. J., Tan, D., Vaughan, J. M., Chang, T., Diedrich, J. K., Andrade, L., Kim, A., Zhang, T., Manor, U., & Saghatelian, A. (2019). Regulation of the ER stress response by a mitochondrial microprotein. Nature Communications, 10, 4883. https://doi.org/10.1038/s41467-019-12816-z
Chu, Q., & Saghatelian, A. (2019). A hidden ORF reveals an immune protector. Biochemistry, 58, 1022–1023. https://doi.org/10.1021/acs.biochem.8b01317
Chugunova, A., Navalayeu, T., Dontsova, O., & Sergiev, P. (2018). Mining for small translated ORFs. Journal of Proteome Research, 17, 1–11. https://doi.org/10.1021/acs.jproteome.7b00707
Clamer, M., Tebaldi, T., Lauria, F., Bernabo, P., Gomez-Biagi, R. F., Marchioretto, M., Kandala, D. T., Minati, L., Perenthaler, E., Gubert, D., Pasquardini, L., Guella, G., Groen, E. J. N., Gillingwater, T. H., Quattrone, A., & Viero, G. (2018). Active ribosome profiling with RiboLace. Cell Reports, 25(1097–1108), e1095. https://doi.org/10.1016/j.celrep.2018.09.084
Cobb, L. J., Lee, C. H., Xiao, J. L., Yen, K., Wong, R. G., Nakamura, H. K., Mehta, H. H., Gao, Q. L., Ashur, C., Huffman, D. M., Wan, J. X., Muzumdar, R., Barzilai, N., & Cohen, P. (2016). Naturally occurring mitochondrial-derived peptides are age-dependent regulators of apoptosis, insulin sensitivity, and inflammatory markers. Aging, 8, 796–809. https://doi.org/10.18632/aging.100943
Couso, J.-P., & Patraquim, P. (2017). Classification and function of small open reading frames. Nature Reviews Molecular Cell Biology, 18, 575–589. https://doi.org/10.1038/nrm.2017.58
Craik, D. J., & Du, J. (2017). Cyclotides as drug design scaffolds. Current Opinion in Chemical Biology, 38, 8–16. https://doi.org/10.1016/j.cbpa.2017.01.018
Crook, Z. R., Nairn, N. W., & Olson, J. M. (2020). Miniproteins as a powerful modality in drug development. Trends in Biochemical Sciences, 45, 332–346. https://doi.org/10.1016/j.tibs.2019.12.008
D’Lima, N. G., Ma, J., Winkler, L., Chu, Q., Loh, K. H., Corpuz, E. O., Budnik, B. A., Lykke-Andersen, J., Saghatelian, A., & Slavoff, S. A. (2017). A human microprotein that interacts with the mRNA decapping complex. Nature Chemical Biology, 13, 174–180. https://doi.org/10.1038/nchembio.2249
Duffield, G. E., Watson, N. P., Mantani, A., Peirson, S. N., Robles-Murguia, M., Loros, J. J., Israel, M. A., & Dunlap, J. C. (2009). A role for Id2 in regulating photic entrainment of the mammalian circadian system. Current Biology, 19, 297–304. https://doi.org/10.1016/j.cub.2008.12.052
Eguen, T., Straub, D., Graeff, M., & Wenkel, S. (2015). MicroProteins: small size-big impact. Trends in Plant Science, 20, 477–482. https://doi.org/10.1016/j.tplants.2015.05.011
Fang, J., Morsalin, S., Rao, V. N., & Reddy, E. S. (2017). Decoding of non-coding DNA and non-coding RNA: pri-micro RNA-encoded novel peptides regulate migration of cancer cells. Journal of Pharmaceutical Sciences and Pharmacology, 3, 23. https://doi.org/10.1166/jpsp.2017.1070
Friesen, M., Warren, C. R., Yu, H. J., Toyohara, T., Ding, Q. R., Florido, M. H. C., Sayre, C., Pope, B. D., Goff, L. A., Rinn, J. L., & Cowan, C. A. (2020). Mitoregulin controls beta-oxidation in human and mouse adipocytes. Stem Cell Reports, 14, 590–602. https://doi.org/10.1016/j.stemcr.2020.03.002
Frith, M. C., Forrest, A. R., Nourbakhsh, E., Pang, K. C., Kai, C., Kawai, J., Carninci, P., Hayashizaki, Y., Bailey, T. L., & Grimmond, S. M. (2006). The abundance of short proteins in the mammalian proteome. PLoS Genetics, 2, e52. https://doi.org/10.1371/journal.pgen.0020052
Galindo, M. I., Pueyo, J. I., Fouix, S., Bishop, S. A., & Couso, J. P. (2007). Peptides encoded by short ORFs control development and define a new eukaryotic gene family. PLoS Biology, 5, e106. https://doi.org/10.1371/journal.pbio.0050106
Gelfand, M. S. (1995). Prediction of function in DNA sequence analysis. Journal of Computational Biology, 2, 87–115. https://doi.org/10.1089/cmb.1995.2.87
Gonzalez, C., Sims, J. S., Hornstein, N., Mela, A., Garcia, F., Lei, L., Gass, D. A., Amendolara, B., Bruce, J. N., Canoll, P., & Sims, P. A. (2014). Ribosome profiling reveals a cell-type-specific translational landscape in brain tumors. The Journal of Neuroscience, 34, 10924–10936. https://doi.org/10.1523/JNEUROSCI.0084-14.2014
Guo, B., Wu, S., Zhu, X., Zhang, L., Deng, J., Li, F., Wang, Y., Zhang, S., Wu, R., Lu, J., & Zhou, Y. (2020). Micropeptide CIP2A-BP encoded by LINC00665 inhibits triple-negative breast cancer progression. The EMBO Journal, 39, e102190. https://doi.org/10.15252/embj.2019102190
Guo, Z. W., Meng, Y., Zhai, X. M., Xie, C., Zhao, N., Li, M., Zhou, C. L., Li, K., Liu, T. C., Yang, X. X., & Wu, Y. S. (2019). Translated long non-coding ribonucleic acid ZFAS1 promotes cancer cell migration by elevating reactive oxygen species production in hepatocellular carcinoma. Frontiers in Genetics, 10, 12. https://doi.org/10.3389/fgene.2019.01111
Hanada, K., Akiyama, K., Sakurai, T., Toyoda, T., Shinozaki, K., & Shiu, S. H. (2010). sORF finder: a program package to identify small open reading frames with high coding potential. Bioinformatics, 26, 399–400. https://doi.org/10.1093/bioinformatics/btp688
Hao, P., Zhang, H., Sze, & S. K. (2011). Application of electrostatic repulsion hydrophilic interaction chromatography to the characterization of proteome, glycoproteome, and phosphoproteome using nano LC–MS/MS. In: S. Toms, R. Weil (Eds.), Nanoproteomics. Methods in molecular biology (Methods and Protocols), (1th ed., pp. 305–318). Humana Press. https://doi.org/10.1007/978-1-61779-319-6_23
Hashimoto, Y., Ito, Y., Niikura, T., Shao, Z., Hata, M., Oyama, F., & Nishimoto, I. (2001). Mechanisms of neuroprotection by a novel rescue factor humanin from Swedish mutant amyloid precursor protein. Biochemical and Biophysical Research Communications, 283, 460–468. https://doi.org/10.1006/bbrc.2001.4765
He, Y. J., Meghani, K., Caron, M. C., Yang, C., Ronato, D. A., Bian, J., Sharma, A., Moore, J., Niraj, J., Detappe, A., Doench, J. G., Legube, G., Root, D. E., D’Andrea, A. D., Drane, P., De, S., Konstantinopoulos, P. A., Masson, J. Y., & Chowdhury, D. (2018). DYNLL1 binds to MRE11 to limit DNA end resection in BRCA1-deficient cells. Nature, 563, 522–526. https://doi.org/10.1038/s41586-018-0670-5
Heiman, M., Kulicke, R., Fenster, R. J., Greengard, P., & Heintz, N. (2014). Cell type-specific mRNA purification by translating ribosome affinity purification (TRAP). Nature Protocols, 9, 1282–1291. https://doi.org/10.1038/nprot.2014.085
Ho, L., Tan, S. Y. X., Wee, S., Wu, Y. X., Tan, S. J. C., Ramakrishna, N. B., Chng, S. C., Nama, S., Szczerbinska, I., Chan, Y. S., Avery, S., Tsuneyoshi, N., Ng, H. H., Gunaratne, J., Dunn, N. R., & Reversade, B. (2015). ELABELA is an endogenous growth factor that sustains hESC self-renewal via the PI3K/AKT pathway. Cell Stem Cell, 17, 435–447. https://doi.org/10.1016/j.stem.2015.08.010
Huang, J. Z., Chen, M., Chen, D. E., Gao, X. C., Zhu, S., Huang, H., Hu, M., Zhu, H., & Yan, G. R. (2017). A peptide encoded by a putative lncRNA HOXB-AS3 suppresses colon cancer growth. Molecular Cell, 68, 171-184 e176. https://doi.org/10.1016/j.molcel.2017.09.015
Hurst, L. D. (2002). The Ka/Ks ratio: diagnosing the form of sequence evolution. Trends in Genetics, 18, 486–487. https://doi.org/10.1016/S01689525(02)027221
Iavarone, A., Garg, P., Lasorella, A., Hsu, J., & Israel, M. A. (1994). The helix-loop-helix protein Id-2 enhances cell-proliferation and binds to the retinoblastoma protein. Genes and Development, 8, 1270–1284. https://doi.org/10.1101/gad.8.11.1270
Ingolia, N. T., Ghaemmaghami, S., Newman, J. R., & Weissman, J. S. (2009). Genome-wide analysis in vivo of translation with nucleotide resolution using ribosome profiling. Science, 324, 218–223. https://doi.org/10.1126/science.1168978
Ingolia, N. T., Lareau, L. F., & Weissman, J. S. (2011). Ribosome profiling of mouse embryonic stem cells reveals the complexity and dynamics of mammalian proteomes. Cell, 147, 789–802. https://doi.org/10.1016/j.cell.2011.10.002
Jackson, R., Kroehling, L., Khitun, A., Bailis, W., Jarret, A., York, A. G., Khan, O. M., Brewer, J. R., Skadow, M. H., Duizer, C., Harman, C. C. D., Chang, L., Bielecki, P., Solis, A. G., Steach, H. R., Slavoff, S., & Flavell, R. A. (2018). The translation of non-canonical open reading frames controls mucosal immunity. Nature, 564, 434–438. https://doi.org/10.1038/s41586-018-0794-7
Jorgensen, R. A., & Dorantes-Acosta, A. E. (2012). Conserved peptide upstream open reading frames are associated with regulatory genes in angiosperms. Frontiers in Plant Science, 3, 191. https://doi.org/10.3389/fpls.2012.00191
Kang, M., Tang, B., Li, J., Zhou, Z., Liu, K., Wang, R., Jiang, Z., Bi, F., Patrick, D., Kim, D., Mitra, A. K., & Yang-Hartwich, Y. (2020). Identification of miPEP133 as a novel tumor-suppressor microprotein encoded by miR-34a pri-miRNA. Molecular Cancer, 19, 143. https://doi.org/10.1186/s12943-020-01248-9
Kastenmayer, J. P., Ni, L., Chu, A., Kitchen, L. E., Au, W. C., Yang, H., Carter, C. D., Wheeler, D., Davis, R. W., Boeke, J. D., Snyder, M. A., & Basrai, M. A. (2006). Functional genomics of genes with small open reading frames (sORFs) in S-cerevisiae. Genome Research, 16, 365–373. https://doi.org/10.1101/gr.4355406
Khitun, A., Ness, T. J., & Slavoff, S. A. (2019). Small open reading frames and cellular stress responses. Molecular Omics, 15, 108–116. https://doi.org/10.1039/c8mo00283e
Khitun, A., & Slavoff, S. A. (2019). Proteomic detection and validation of translated small open reading frames. Current Protocols in Chemical Biology, 11, e77. https://doi.org/10.1002/cpch.77
Kim, S. J., Xiao, J. L., Wan, J. X., Cohen, P., & Yen, K. (2017). Mitochondrially derived peptides as novel regulators of metabolism. Journal of Physiology, 595, 6613–6621. https://doi.org/10.1113/Jp274472
Kondo, T., Hashimoto, Y., Kato, K., Inagaki, S., Hayashi, S., & Kageyama, Y. (2007). Small peptide regulators of actin-based cell morphogenesis encoded by a polycistronic mRNA. Nature Cell Biology, 9, 660–665. https://doi.org/10.1038/ncb1595
Kondo, T., Plaza, S., Zanet, J., Benrabah, E., Valenti, P., Hashimoto, Y., Kobayashi, S., Payre, F., & Kageyama, Y. (2010). Small peptides switch the transcriptional activity of shavenbaby during drosophila embryogenesis. Science, 329, 336–339. https://doi.org/10.1126/science.1188158
Koster, J., & Plasterk, R. H. A. (2019). A library of Neo Open Reading Frame peptides (NOPs) as a sustainable resource of common neoantigens in up to 50% of cancer patients. Scientific Reports, 9, 6577. https://doi.org/10.1038/s41598-019-42729-2
Lee, C., Zeng, J., Drew, B. G., Sallam, T., Martin-Montalvo, A., Wan, J., Kim, S. J., Mehta, H., Hevener, A. L., de Cabo, R., & Cohen, P. (2015). The mitochondrial-derived peptide MOTS-c promotes metabolic homeostasis and reduces obesity and insulin resistance. Cell Metabolism, 21, 443–454. https://doi.org/10.1016/j.cmet.2015.02.009
Lee, Y. D., Wang, J., Stubbe, J., & Elledge, S. J. (2008). Dif1 is a DNA-damage-regulated facilitator of nuclear import for ribonucleotide reductase. Molecular Cell, 32, 70–80. https://doi.org/10.1016/j.molcel.2008.08.018
Li, H., Xiao, L., Zhang, L. L., Wu, J. R., Wei, B., Sun, N. H., & Zhao, Y. (2018). FSPP: a tool for genome-wide prediction of smORF-encoded peptides and their functions. Frontiers in Genetics, 9, 8. https://doi.org/10.3389/fgene.2018.00096
Li, M. W., Li, X., Zhang, Y. N., Wu, H. M., Zhou, H. Z., Ding, X., Zhang, X. M., Jin, X. R., Wang, Y., Yin, X. Q., Li, C. C., Yang, P. W., & Xu, H. M. (2020a). Micropeptide MIAC inhibits HNSCC progression by interacting with aquaporin 2. Journal of the American Chemical Society, 142, 6708–6716. https://doi.org/10.1021/jacs.0c00706
Li, X., Jin, X., Sharma, S., Liu, X., Zhang, J., Niu, Y., Li, J., Li, Z., Zhang, J., Cao, Q., Hou, W., Du, L. L., Liu, B., & Lou, H. (2019). Mck1 defines a key S-phase checkpoint effector in response to various degrees of replication threats. PLoS Genetics, 15, e1008136. https://doi.org/10.1371/journal.pgen.1008136
Li, X. L., Pongor, L., Tang, W., Das, S., Muys, B. R., Jones, M. F., Lazar, S. B., Dangelmaier, E. A., Hartford, C. C., Grammatikakis, I., Hao, Q., Sun, Q., Schetter, A., Martindale, J. L., Tang, B., Jenkins, L. M., Robles, A. I., Walker, R. L., Ambs, S., et al. (2020b). A small protein encoded by a putative lncRNA regulates apoptosis and tumorigenicity in human colorectal cancer cells. eLife, 9, e53734. https://doi.org/10.7554/eLife.53734
Lin, M. F., Jungreis, I., & Kellis, M. (2011). PhyloCSF: a comparative genomics method to distinguish protein coding and non-coding regions. Bioinformatics, 27, i275-282. https://doi.org/10.1093/bioinformatics/btr209
Lin, Y. F., Xiao, M. H., Chen, H. X., Meng, Y., Zhao, N., Yang, L., Tang, H., Wang, J. L., Liu, X., Zhu, Y., & Zhuang, S. M. (2019). A novel mitochondrial micropeptide MPM enhances mitochondrial respiratory activity and promotes myogenic differentiation. Cell Death & Disease, 10, 528. https://doi.org/10.1038/s41419-019-1767-y
Lu, S. H., Zhang, J., Lian, X. L., Sun, L., Meng, K., Chen, Y., Sun, Z. H., Yin, X. F., Li, Y. X., Zhao, J., Wang, T., Zhang, G., & He, Q. Y. (2019). A hidden human proteome encoded by “non-coding” genes. Nucleic Acids Research, 47, 8111–8125. https://doi.org/10.1093/nar/gkz646
Luo, W. S., Grupp, I. L., Harrer, J., Ponniah, S., Grupp, G., Duffy, J. J., Doetschman, T., & Kranias, E. G. (1994). Targeted ablation of the phospholamban gene is associated with markedly enhanced myocardial-contractility and loss of beta-agonist stimulation. Circulation Research, 75, 401–409. https://doi.org/10.1161/01.RES.75.3.401
Ma, J., Diedrich, J. K., Jungreis, I., Donaldson, C., Vaughan, J., Kellis, M., Yates, J. R., 3rd., & Saghatelian, A. (2016). Improved identification and analysis of small open reading frame encoded polypeptides. Analytical Chemistry, 88, 3967–3975. https://doi.org/10.1021/acs.analchem.6b00191
Ma, J., Ward, C. C., Jungreis, I., Slavoff, S. A., Schwaid, A. G., Neveu, J., Budnik, B. A., Kellis, M., & Saghatelian, A. (2014). Discovery of human sORF-encoded polypeptides (SEPs) in cell lines and tissue. Journal of Proteome Research, 13, 1757–1765. https://doi.org/10.1021/pr401280w
Magnani, E., de Klein, N., Nam, H. I., Kim, J. G., Pham, K., Fiume, E., Mudgett, M. B., & Rhee, S. Y. (2014). A comprehensive analysis of microProteins reveals their potentially widespread mechanism of transcriptional regulation. Plant Physiology, 165, 149–159. https://doi.org/10.1104/pp.114.235903
Magny, E. G., Pueyo, J. I., Pearl, F. M. G., Cespedes, M. A., Niven, J. E., Bishop, S. A., & Couso, J. P. (2013). Conserved regulation of cardiac calcium uptake by peptides encoded in small open reading frames. Science, 341, 1116–1120. https://doi.org/10.1126/science.1238802
Makarewich, C. A. (2020). The hidden world of membrane microproteins. Experimental Cell Research, 388, 111853. https://doi.org/10.1016/j.yexcr.2020.111853
Makarewich, C. A., Baskin, K. K., Munir, A. Z., Bezprozvannaya, S., Sharma, G., Khemtong, C., Shah, A. M., McAnally, J. R., Malloy, C. R., Szweda, L. I., Bassel-Duby, R., & Olson, E. N. (2018). MOXI is a mitochondrial micropeptide that enhances fatty acid beta-oxidation. Cell Reports, 23, 3701–3709. https://doi.org/10.1016/j.celrep.2018.05.058
Makarewich, C. A., & Olson, E. N. (2017). Mining for micropeptides. Trends in Cell Biology, 27, 685–696. https://doi.org/10.1016/j.tcb.2017.04.006
Matsumoto, A., Pasut, A., Matsumoto, M., Yamashita, R., Fung, J., Monteleone, E., Saghatelian, A., Nakayama, K. I., Clohessy, J. G., & Pandolfi, P. P. (2017). mTORC1 and muscle regeneration are regulated by the LINC00961-encoded SPAR polypeptide. Nature, 541, 228–232. https://doi.org/10.1038/nature21034
Meng, N., Chen, M., Chen, D., Chen, X. H., Wang, J. Z., Zhu, S., He, Y. T., Zhang, X. L., Lu, R. X., & Yan, G. R. (2020). Small protein hidden in lncRNA LOC90024 promotes “cancerous” RNA splicing and tumorigenesis. Advanced Science, 7, 1903233. https://doi.org/10.1002/advs.201903233
Merino-Valverde, I., Greco, E., & Abad, M. (2020). The microproteome of cancer: From invisibility to relevance. Experimental Cell Research, 392, 111997. https://doi.org/10.1016/j.yexcr.2020.111997
Meurisse, J., Bacquin, A., Richet, N., Charbonnier, J. B., Ochsenbein, F., & Peyroche, A. (2014). Hug1 is an intrinsically disordered protein that inhibits ribonucleotide reductase activity by directly binding Rnr2 subunit. Nucleic Acids Research, 42, 13174–13185. https://doi.org/10.1093/nar/gku1095
Min, K. W., Davila, S., Zealy, R. W., Lloyd, L. T., Lee, I. Y., Lee, R., Roh, K. H., Jung, A., Jemielity, J., Choi, E. J., Chang, J. H., & Yoon, J. H. (2017). eIF4E phosphorylation by MST1 reduces translation of a subset of mRNAs, but increases lncRNA translation. Biochimica Et Biophysica Acta Gene Regulatory Mechanisms, 1860, 761–772. https://doi.org/10.1016/j.bbagrm.2017.05.002
Nelson, B. R., Makarewich, C. A., Anderson, D. M., Winders, B. R., Troupes, C. D., Wu, F., Reese, A. L., McAnally, J. R., Chen, X., Kavalali, E. T., Cannon, S. C., Houser, S. R., Bassel-Duby, R., & Olson, E. N. (2016). A peptide encoded by a transcript annotated as long noncoding RNA enhances SERCA activity in muscle. Science, 351, 271–275. https://doi.org/10.1126/science.aad4076
Nguyen, H. L., Yang, X., & Omiecinski, C. J. (2013). Expression of a novel mRNA transcript for human microsomal epoxide hydrolase (EPHX1) is regulated by short open reading frames within its 5’-untranslated region. RNA, 19, 752–766. https://doi.org/10.1261/rna.037036.112
Odermatt, A., Taschner, P. E., Scherer, S. W., Beatty, B., Khanna, V. K., Cornblath, D. R., Chaudhry, V., Yee, W. C., Schrank, B., Karpati, G., Breuning, M. H., Knoers, N., & MacLennan, D. H. (1997). Characterization of the gene encoding human sarcolipin (SLN), a proteolipid associated with SERCA1: absence of structural mutations in five patients with Brody disease. Genomics, 45, 541–553. https://doi.org/10.1006/geno.1997.4967
Orr, M. W., Mao, Y., Storz, G., & Qian, S. B. (2020). Alternative ORFs and small ORFs: shedding light on the dark proteome. Nucleic Acids Research, 48, 1029–1042. https://doi.org/10.1093/nar/gkz734
Oyama, M., Itagaki, C., Hata, H., Suzuki, Y., Izumi, T., Natsume, T., Isobe, T., & Sugano, S. (2004). Analysis of small human proteins reveals the translation of upstream open reading frames of mRNAs. Genome Research, 14, 2048–2052. https://doi.org/10.1101/gr.2384604
Pang, Y. N., Liu, Z. Y., Han, H., Wang, B. L., Li, W., Mao, C. B., & Liu, S. R. (2020). Peptide SMIM30 promotes HCC development by inducing SRC/YES1 membrane anchoring and MAPK pathway activation. Journal of Hepatology, 73, 1155–1169. https://doi.org/10.1016/j.jhep.2020.05.028
Peeters, M. K. R., & Menschaert, G. (2020). The hunt for sORFs: A multidisciplinary strategy. Experimental Cell Research, 391, 111923. https://doi.org/10.1016/j.yexcr.2020.111923
Plaza, S., Menschaert, G., & Payre, F. (2017). In search of lost small peptides. Annual Review of Cell and Developmental Biology, 33, 391–416. https://doi.org/10.1146/annurev-cellbio-100616-060516
Polycarpou-Schwarz, M., Gross, M., Mestdagh, P., Schott, J., Grund, S. E., Hildenbrand, C., Rom, J., Aulmann, S., Sinn, H. P., Vandesompele, J., & Diederichs, S. (2018). The cancer-associated microprotein CASIMO1 controls cell proliferation and interacts with squalene epoxidase modulating lipid droplet formation. Oncogene, 37, 4750–4768. https://doi.org/10.1038/s41388-018-0281-5
Pueyo, J. I., Magny, E. G., & Couso, J. P. (2016). New peptides under the s(ORF)ace of the genome. Trends in Biochemical Sciences, 41, 665–678. https://doi.org/10.1016/j.tibs.2016.05.003
Raj, A., Wang, S. H., Shim, H., Harpak, A., Li, Y. I., Engelmann, B., Stephens, M., Gilad, Y., & Pritchard, J. K. (2016). Thousands of novel translated open reading frames in humans inferred by ribosome footprint profiling. eLife, 5, e13328. https://doi.org/10.7554/eLife.13328
Rapali, P., Szenes, A., Radnai, L., Bakos, A., Pal, G., & Nyitray, L. (2011). DYNLL/LC8: a light chain subunit of the dynein motor complex and beyond. The FEBS Journal, 278, 2980–2996. https://doi.org/10.1111/j.1742-4658.2011.08254.x
Rathore, A., Martinez, T. F., Chu, Q., & Saghatelian, A. (2018). Small, but mighty? Searching for human microproteins and their potential for understanding health and disease. Expert Review of Proteomics, 15, 963–965. https://doi.org/10.1080/14789450.2018.1547194
Renz, P. F., Valdivia-Francia, F., & Sendoel, A. (2020). Some like it translated: small ORFs in the 5’ UTR. Experimental Cell Research, 396, 112229. https://doi.org/10.1016/j.yexcr.2020.112229
Roschger, C., & Cabrele, C. (2017). The Id-protein family in developmental and cancer-associated pathways. Cell Communication and Signaling, 15, 7. https://doi.org/10.1186/s12964-016-0161-y
Ruzinova, M. B., & Benezra, R. (2003). Id proteins in development, cell cycle and cancer. Trends in Cell Biology, 13, 410–418. https://doi.org/10.1016/s0962-8924(03)00147-8
Saghatelian, A., & Couso, J. P. (2015). Discovery and characterization of smORF-encoded bioactive polypeptides. Nature Chemical Biology, 11, 909–916. https://doi.org/10.1038/nchembio.1964
Savard, J., Marques-Souza, H., Aranda, M., & Tautz, D. (2006). A segmentation gene in Tribolium produces a polycistronic mRNA that codes for multiple conserved peptides. Cell, 126, 559–569. https://doi.org/10.1016/j.cell.2006.05.053
Schlesinger, D., & Elsasser, S. J. (2021). Revisiting sORFs: overcoming challenges to identify and characterize functional microproteins. The FEBS Journal. https://doi.org/10.1111/febs.15769
Slavoff, S. A., Heo, J., Budnik, B. A., Hanakahi, L. A., & Saghatelian, A. (2014). A human short open reading frame (sORF)-encoded polypeptide that stimulates DNA end joining. The Journal of Biological Chemistry, 289, 10950–10957. https://doi.org/10.1074/jbc.C113.533968
Slavoff, S. A., Mitchell, A. J., Schwaid, A. G., Cabili, M. N., Ma, J., Levin, J. Z., Karger, A. D., Budnik, B. A., Rinn, J. L., & Saghatelian, A. (2013). Peptidomic discovery of short open reading frame-encoded peptides in human cells. Nature Chemical Biology, 9, 59–64. https://doi.org/10.1038/nchembio.1120
Sousa, M. E., & Farkas, M. H. (2018). Micropeptide. PLoS Genetics, 14, e1007764. https://doi.org/10.1371/journal.pgen.1007764
Starck, S. R., Tsai, J. C., Chen, K., Shodiya, M., Wang, L., Yahiro, K., Martins-Green, M., Shastri, N., & Walter, P. (2016). Translation from the 5’ untranslated region shapes the integrated stress response. Science, 351, aad3867. https://doi.org/10.1126/science.aad3867
Staudt, A. C., & Wenkel, S. (2011). Regulation of protein function by “microProteins.” EMBO Reports, 12, 35–42. https://doi.org/10.1038/embor.2010.196
Stein, C. S., Jadiya, P., Zhang, X. M., McLendon, J. M., Abouassaly, G. M., Witmer, N. H., Anderson, E. J., Elrod, J. W., & Boudreau, R. L. (2018). Mitoregulin: a lncRNA-encoded microprotein that supports mitochondrial supercomplexes and respiratory efficiency. Cell Reports, 23, 3710–3720
Storz, G., Wolf, Y. I., & Ramamurthi, K. S. (2014). Small proteins can no longer be ignored. Annual Review of Biochemistry, 83, 753–777. https://doi.org/10.1146/annurev-biochem-070611-102400
Vanderperre, B., Lucier, J. F., Bissonnette, C., Motard, J., Tremblay, G., Vanderperre, S., Wisztorski, M., Salzet, M., Boisvert, F. M., & Roucou, X. (2013). Direct detection of alternative open reading frames translation products in human significantly expands the proteome. PLoS ONE, 8, e70698. https://doi.org/10.1371/journal.pone.0070698
Vitorino, R., Guedes, S., Amado, F., Santos, M., & Akimitsu, N. (2021). The role of micropeptides in biology. Cellular and Molecular Life Sciences. https://doi.org/10.1007/s00018-020-03740-3
Wang, J., Zhu, S., Meng, N., He, Y., Lu, R., & Yan, G. R. (2019). ncRNA-encoded peptides or proteins and cancer. Molecular Therapy, 27, 1718–1725. https://doi.org/10.1016/j.ymthe.2019.09.001
Wu, P., Mo, Y., Peng, M., Tang, T., Zhong, Y., Deng, X., Xiong, F., Guo, C., Wu, X., Li, Y., Li, X., Li, G., Zeng, Z., & Xiong, W. (2020a). Emerging role of tumor-related functional peptides encoded by lncRNA and circRNA. Molecular Cancer, 19, 22. https://doi.org/10.1186/s12943-020-1147-3
Wu, S., Zhang, L., Deng, J., Guo, B., Li, F., Wang, Y., Wu, R., Zhang, S., Lu, J., & Zhou, Y. (2020b). A Novel micropeptide encoded by Y-Linked LINC00278 links cigarette smoking and AR signaling in male esophageal squamous cell carcinoma. Cancer Research, 80, 2790–2803. https://doi.org/10.1158/0008-5472.CAN-19-3440
Wu, X., & Huang, M. (2008). Dif1 controls subcellular localization of ribonucleotide reductase by mediating nuclear import of the R2 subunit. Molecular and Cell Biology, 28, 7156–7167 https://doi.org/10.1128/MCB.01388-08
Xia, X., Li, X. X., Li, F. Y., Wu, X. J., Zhang, M. L., Zhou, H. K., Huang, N. N., Yang, X. S., Xiao, F. Z., Liu, D. W., Yang, L. X., & Zhang, N. (2019). A novel tumor suppressor protein encoded by circular AKT3 RNA inhibits glioblastoma tumorigenicity by competing with active phosphoinositide-dependent Kinase-1. Molecular Cancer, 18, 131. https://doi.org/10.1186/s12943-019-1083-2
Xiao, Z. T., Huang, R. Y., Xing, X. D., Chen, Y. L., Deng, H. T., & Yang, X. R. (2018). De novo annotation and characterization of the translatome with ribosome profiling data. Nucleic Acids Research, 46, e61. https://doi.org/10.1093/nar/gky179
Xu, W. L., Deng, B., Lin, P. H., Liu, C., Li, B., Huang, Q. J., Zhou, H., Yang, J. H., & Qu, L. H. (2020). Ribosome profiling analysis identified a KRAS-interacting microprotein that represses oncogenic signaling in hepatocellular carcinoma cells. Science China-Life Sciences, 63, 529–542. https://doi.org/10.1007/s11427-019-9580-5
Yang, X., Tschaplinski, T. J., Hurst, G. B., Jawdy, S. S., Abraham, P. E., Lankford, P. K., Adams, R. M., Shah, M. B., Hettich, R. L., Lindquist, E., Kalluri, U.C., Gunter, L.E., Pennacchio, C., & Tuskan GA. (2011). Discovery and annotation of small proteins using genomics, proteomics, and computational approaches. Genome Research, 21, 634–641. https://doi.org/10.1101/gr.109280.110
Yang, Y., Gao, X., Zhang, M., Yan, S., Sun, C., Xiao, F., Huang, N., Yang, X., Zhao, K., Zhou, H., Huang, S., Xie, B., & Zhang, N. (2018). Novel role of FBXW7 circular RNA in repressing glioma tumorigenesis. Journal of the National Cancer Institute. https://doi.org/10.1093/jnci/djx166
Yin, X. Q., Jing, Y. Y., & Xu, H. M. (2019). Mining for missed sORF-encoded peptides. Expert Review of Proteomics, 16, 257–266. https://doi.org/10.1080/14789450.2019.1571919
Zanet, J., Chanut-Delalande, H., Plaza, S., & Payre, F. (2016). Small peptides as newcomers in the control of drosophila development. Current Topics in Developmental Biology, 117, 199–219. https://doi.org/10.1016/bs.ctdb.2015.11.004
Zhang, H., Wang, Y. R., Wu, X. K., Tang, X. L., Wu, C. C., & Lu, J. (2021). Determinants of genome-wide distribution and evolution of uORFs in eukaryotes. Nature Communications, 12, 1076. https://doi.org/10.1038/s41467-021-21394-y
Zhang, M., Huang, N., Yang, X., Luo, J., Yan, S., Xiao, F., Chen, W., Gao, X., Zhao, K., Zhou, H., Li, Z., Ming, L., Xie, B., & Zhang, N. (2018a). A novel protein encoded by the circular form of the SHPRH gene suppresses glioma tumorigenesis. Oncogene, 37, 1805–1814. https://doi.org/10.1038/s41388-017-0019-9
Zhang, M., Zhao, K., Xu, X., Yang, Y., Yan, S., Wei, P., Liu, H., Xu, J., Xiao, F., Zhou, H., Yang, X., Huang, N., Liu, J., He, K., Xie, K., Zhang, G., Huang, S., & Zhang, N. (2018b). A peptide encoded by circular form of LINC-PINT suppresses oncogenic transcriptional elongation in glioblastoma. Nature Communications, 9, 4475. https://doi.org/10.1038/s41467-018-06862-2
Zhang, Q., Vashisht, A. A., O’Rourke, J., Corbel, S. Y., Moran, R., Romero, A., Miraglia, L., Zhang, J., Durrant, E., Schmedt, C., Sampath, S. C., & Sampath, S. C. (2017). The microprotein Minion controls cell fusion and muscle formation. Nature Communications, 8, 15664. https://doi.org/10.1038/ncomms15664
Zhang, S., Reljic, B., Liang, C., Kerouanton, B., Francisco, J. C., Peh, J. H., Mary, C., Jagannathan, N. S., Olexiouk, V., Tang, C., Fidelito, G., Nama, S., Cheng, R. K., Wee, C. L., Wang, L. C., Roggli, P. D., Sampath, P., Lane, L., Petretto, E., et al. (2020). Mitochondrial peptide BRAWNIN is essential for vertebrate respiratory complex III assembly. Nature Communications, 11, 1312. https://doi.org/10.1038/s41467-020-14999-2
Zhang, Y., Fonslow, B. R., Shan, B., Baek, M. C., & Yates, J. R., 3rd. (2013). Protein analysis by shotgun/bottom-up proteomics. Chemical Reviews, 113, 2343–2394. https://doi.org/10.1021/cr3003533
Zhao, J. W., Lee, E. E., Kim, J., Yang, R., Chamseddin, B., Ni, C. Y., Gusho, E., Xie, Y., Chiang, C. M., Buszczak, M., Zhan, X. W., Laimins, L., & Wang, R. C. (2019). Transforming activity of an oncoprotein-encoding circular RNA from human papillomavirus. Nature Communications, 10, 2300. https://doi.org/10.1038/s41467-019-10246-5
Zhao, X., Chabes, A., Domkin, V., Thelander, L., & Rothstein, R. (2001). The ribonucleotide reductase inhibitor Sml1 is a new target of the Mec1-Rad53 kinase cascade during growth and in response to DNA damage. The EMBO Journal, 20, 3544–3553. https://doi.org/10.1093/emboj/20.13.3544
Zheng, X., Chen, L. J., Zhou, Y., Wang, Q., Zheng, Z. J., Xu, B., Wu, C., Zhou, Q., Hu, W. W., Wu, C. P., & Jiang, J. T. (2019). A novel protein encoded by a circular RNA circPPP1R12A promotes tumor pathogenesis and metastasis of colon cancer via Hippo-YAP signaling. Molecular Cancer, 18, 47. https://doi.org/10.1186/s12943-019-1010-6
Zhi, X., Zhang, J., Cheng, Z., Bian, L., & Qin, J. (2019). circLgr4 drives colorectal tumorigenesis and invasion through Lgr4-targeting peptide. International Journal of Cancer. https://doi.org/10.1002/ijc.32549
Zhou, B., Yang, H., Yang, C., Bao, Y. L., Yang, S. M., Liu, J., & Xiao, Y. F. (2021). Translation of noncoding RNAs and cancer. Cancer Letters, 497, 89–99. https://doi.org/10.1016/j.canlet.2020.10.002
Zhu, S., Wang, J. Z., Chen, D., He, Y. T., Meng, N., Chen, M., Lu, R. X., Chen, X. H., Zhang, X. L., & Yan, G. R. (2020). An oncopeptide regulates m(6)A recognition by the m(6)A reader IGF2BP1 and tumorigenesis. Nature Communications, 11, 1685. https://doi.org/10.1038/s41467-020-15403-9
Acknowledgements
We thank Dr. Lili Li for help in figure preparation. This work was funded by the Beijing Municipal Natural Science Foundation (Grant no. 5204036); National Key R&D Program of China (Grant no. 2019YFA0903900); National Natural Science Foundation of China (Grant no. 31630005, 31770084).
Author information
Affiliations
Guangdong Key Laboratory for Genome Stability & Disease Prevention, Shenzhen University School of Medicine, Shenzhen, 518060, China
Meiqian Jiang, Huiqiang Lou & Wenya Hou
Corresponding author
Correspondence to Wenya Hou.
Rights and permissions
About this article
Cite this article
Jiang, M., Lou, H. & Hou, W. Microproteins: from behind the scenes to the spotlight. GENOME INSTAB. DIS. 2, 225–239 (2021). https://doi.org/10.1007/s42764-021-00040-3
Received01 April 2021
Revised26 April 2021
Accepted04 May 2021
Published16 May 2021
Issue DateAugust 2021
Share this article
Anyone you share the following link with will be able to read this content:
Get shareable linkKeywords
Microproteins
Small open reading frames
Genome stability
DNA repair
DNA replication
Cancer
用户登录
还没有账号?
立即注册