Genotoxicity testing and recent advances
Review Article
Genome Instability & Disease (2021)
Abstract
Genotoxicity testing, a technique which is used to identify chemicals that cause genetic alterations, includes tests that aid determination of irreversible genetic alterations, as well as those that provide indirect evidence of DNA damage. A panel of tests is commonly considered to comprehensively evaluate the ability of a chemical to induce genotoxicity, since individual tests do not provide information on all end points. Although a standard panel for genotoxicity testing has been considered traditionally for decades, changes based on novel and emerging methodologies have been introduced. The present review provides a brief introduction to the commonly used basic tests intended for regulatory purposes, advanced genotoxicity testing including cells used in genotoxicity testing, application of quantitative structure activity relationship (QSAR), representative genotoxicity high-throughput in vitro assays, and high-dimensional/high information content assays, with specific reference to the application of next-generation sequencing (NGS), which enable genome-wide analyses of mutations in non-cancer cells, thereby revealing the effects of various environmental mutagens.
Preamble
Genotoxicity testing, a technique which helps identify chemicals that cause genetic alterations, includes tests for mutagenicity and DNA damage. Mutagenicity refers to the capability of a substance to induce a genetic mutation, an irreversible event that alters DNA and/or chromosomal number or structure, and may be passed onto subsequent cell generations. Mutations in somatic cells may cause cancer, while mutations in germ cells may be passed on to offspring. DNA damage may not result in permanent alterations since it may be reversed by a DNA repair process. However, accumulation of DNA damage in somatic cells is associated with the development of certain degenerative conditions, such as accelerated aging, immune dysfunction, cardiovascular and neurodegenerative diseases, while such damage in germ cells may be associated with spontaneous abortions, infertility, malformation, or heritable mutations in the offspring. Therefore, genotoxicity testing aids the determination of not only irreversible genetic alteration, but also helps evaluate indirect evidence of mutations, such as DNA strand breaks and DNA adduct formation. A combination of multiple tests (usually referred to as a test battery) is commonly used to comprehensively evaluate the ability of a chemical to induce genotoxicity, because an individual test does not provide information on all end points. Although a standard genotoxicity test battery has been traditionally used for decades, changes based on novel and emerging methodologies have been introduced. Along with an updated understanding of DNA and chromosome function, interdisciplinarity among computational toxicology, analytical chemistry, and bioinformatics has facilitated a change in genotoxicity testing strategy, which has fundamentally changed the practice of genotoxicity testing. The present article presents a brief introduction to the basic tests stipulated for regulatory purposes, especially those that are commonly used and included in guidelines. Additionally, the present article reviews emerging technologies and discusses prospects for future development and application of genotoxicity testing.
Traditional genotoxicity testing
Several genotoxicity testing techniques that help detect the genotoxicity of chemical substances have been developed using various model organisms. Currently, the Organisation for Economic Co-operation and Development (OECD) guidelines are followed for over 60 toxicity testing methods that help evaluate the effect of chemical substances on human health. Thirteen of such methods are considered to be genotoxicity tests (Table 1); (OECD, 2010) Genotoxicity tests are classified into in vitro tests, which use bacterial cells, cultured mammalian cells, and so on, and in vivo tests, which use animal models, such as mice and rats. These tests are often conducted as screening tests for genotoxicity-based carcinogenicity, mainly in somatic cells. Certain in vivo tests use sperm and/or testes as the target to screen the ability of a substance to induce a hereditary disease via germ cells.
In vitro tests are generally simple and less expensive and provide results in a relatively short term. However, because bacterial and cultured mammalian cells used in in vitro tests usually lack any activity of drug-metabolizing enzymes, such as cytochrome P450 (CYP), these tests are not effective for analysing chemical substances, such as benzo[a]pyrene and aflatoxin, the metabolites of which exhibit genotoxicity. Therefore, where these tests are used to detect the genotoxicity of chemical substances and their metabolites, a 9000 × g centrifugation-derived supernatant of liver homogenate (S9) is added to the sample (ICH, 2011). In several cases, S9 is prepared using samples obtained from rats that have been treated with drug-metabolising enzyme inducers (a combination of phenobarbital and 5,6-benzoflavone, among others). However, in certain instances, the test is conducted using S9 derived from other rodents or humans, depending on the objective. Before use, S9 is subjected to treatment with a cofactor involved in the electron transport chain, such as NADPH, whereupon this mixture of S9 and the cofactor is termed S9 mix. Rather than using S9 mix, microbial strains and cultured mammalian cells transfected with multiple cloned human CYP genes have also been used for in vitro genotoxicity tests. Additionally, a few in vitro tests use primary hepatocytes with residual drug-metabolising enzyme activity in certain instances.
Compared to in vitro tests, in vivo tests provide results that reflect the metabolism, absorption, discharge, and distribution of chemical substances; thus, they present with increased applicability in the assessment of risks posed by such substances to humans, compared with in vitro tests in which the results are investigated after extrapolation. In cases where systemic exposure is considered, these tests are usually conducted using bone marrow and/or peripheral blood as sample tissues. However, when carcinogenicity is suspected in a specific organ or tissue, it is preferable to include and investigate other specific tissues, depending on the chemical involved (e.g. cosmetics), because the occurrence of sufficient exposure to exert effects on the bone marrow and/or peripheral blood is unlikely.
As individual genotoxicity tests have been optimised for use in a specific species and for the detection of a specific end point, a specific test cannot be used to detect all genotoxicities. Therefore, genotoxicity tests are conducted via a combination of various complementary tests for the detection of a wide range of potential genotoxicities of chemical substances. In 2012, the International Council of Harmonization of Technical Requirements for Registration of Pharmaceuticals for Human Use (ICH) established a “Guidance on Genotoxicity Testing and Data Interpretation for Pharmaceuticals Intended for Human Use (S2(R1)).” In this guidance, two approaches have been provided as standard combinations of genotoxicity tests (ICH, 2011).
The first approach is as follows:
1.
A bacterial reverse mutation assay (Ames test) (in vitro test).
2.
A chromosome aberration test, micronucleus test, or mouse lymphoma test using mammalian cells (in vitro test).
3.
A micronucleus test or chromosome aberration test targeting a hematopoietic tissue of rodents (in vivo test).
The second approach is as follows:
1.
An Ames test (in vitro test).
2.
A micronucleus test or chromosome aberration test targeting a hematopoietic tissue of rodents (in vivo test).
3.
A DNA strand break assay (comet assay or alkaline elution assay), a transgenic rodent gene mutation assay, or a UDS test targeting liver of rodents (in vivo tests).
In vitro tests
Bacterial reverse mutation assay (Ames tes)(TG471)
The basic structure and function of DNA or the mutation induction mechanism in bacteria is considered to be shared across higher animals. Mutagenicity testing methods that use bacteria have the following advantages: the results can be more easily interpreted than those in mammalian tests because of advances in the study of DNA damage and its repair mechanisms in bacteria. These testing methods use simpler procedures than those for higher organisms, allowing many samples to be tested over a short time period at a low cost; and databases are readily available because many tests have already been conducted. The most widely used testing method is the bacterial reverse mutation test (Ames test). This test detects a mutagen by using the reverse mutation of an amino acid-requiring strain of Salmonella typhimurium or Escherichia coli as an indicator. Dr. Bruce Ames developed the testing method that uses Salmonella; however, the name is also used in a broader sense for the method that uses E. coli (Maron & Ames, 1983; Mortelmans & Riccio, 2000; Mortelmans & Zeiger, 2000) (Table 2).
All Salmonella strains used for this test carry a mutation in a gene (hisG; hisD) that encodes an enzyme involved in histidine biosynthesis, thus inhibiting the growth of these strains on media that do not contain histidine (histidine requirement). However, another mutation in the relevant gene caused by a chemical allows the synthesis of histidine and formation of colonies by these strains on media that do not contain histidine (histidine non-requirement). As this involves the reversion of the phenotype of the bacterial strain from histidine requirement to histidine non-requirement, it is termed a reverse mutation test. This test may be performed to detect several mutations, because reversion of the phenotype may result not only from re-mutation of the original mutation site, but also from a mutation around this site or in a tRNA gene. The Salmonella strains involved in the Ames test include TA1535, TA100, and TA102 and are used to detect base substitution mutations; additionally, the strains TA1538, TA98, TA1537, and TA97 are used to detect frameshift mutations. All such bacterial strains are highly permeable to chemicals due to a lack of ability to biosynthesise cell wall lipopolysaccharides. Furthermore, all strains, except TA102, display higher sensitivity to mutagens due to deletion of uvrB, an important genetic element which regulates nucleotide excision repair. Furthermore, TA97, TA98, TA100, and TA102 harbour the pKM101 plasmid that enables efficient conversion of DNA damage into a mutation. In the case of E. coli, the tryptophan-requiring mutant WP2uvrA or WP2uvrA/pKM101 is used. The principle and method of the test are the same as when Salmonella is used, except that the required amino acid is tryptophan instead of histidine. These strains are highly sensitive to mutagens that cause base pair substitution and are also sensitive to UV because of their deficiency in the uvrA gene.
The Ames test can be used to screen a wide range of mutagens by using a combination of Salmonella and E. coli with distinct characteristics. In addition, the background mutation frequency of the Ames test is relatively low and stable, allowing a wide dynamic range to be detected between this background mutation frequency and the maximum mutation frequency that is usually detected (Table 2). This wide dynamic range and stable background data allow us to detect even chemicals that induce a weak response with high sensitivity and reliability.
Gene mutation tests using mammalian cells (TG476, 490)
Gene mutation tests using cultured mammalian cells mainly involve the use of a recessive mutation of an endogenous drug-resistance marker gene (HPRT or TK) as an indicator. In contrast with the Ames test, which is a reverse mutation test, the mutation test involving these genes is termed a forward mutation test. This test aids the detection of various types of mutations that lead to the loss or inactivation of proteins encoded by these genes. HPRT, located on the X chromosome, encodes hypoxanthine phosphoribosyltransferase, an enzyme which regulates the salvage cycle of purine bases. The loss of activity of this enzyme in cells results in the development of resistance to 6-thioguanine (6-TG), a DNA synthesis inhibitor that exerts a toxic effect via nucleic acid metabolism. The test involves the subjection of cells to treatment with a test chemical and the subsequent culture of cells on a medium containing 6-TG. The evaluation of mutagenicity of the chemical is based on the number of cell colonies that present with 6-TG resistance development due to the mutation. Not only established cell lines but also primary cultured cells, including human lymphocytes, may be used for conducting this test, only if the cells are diploid cells with an active copy of the X chromosome. Usually, Chinese hamster cell lines, such as CHO, CHL, and V79, are used. This test mainly aids the detection of point mutations, relatively small deletions, insertions in HPRT, and deletions below 3 Mb across the entirety of HPRT. Considerable deletions of essential genes (EGs) that are important for cellular survival may occur at the chromosomal level in the X chromosome, in conjunction with the deletion of genes in the vicinity of HPRT. However, these deletions cannot be detected because they are lethal for the cells (Fig. 1a).
Fig. 1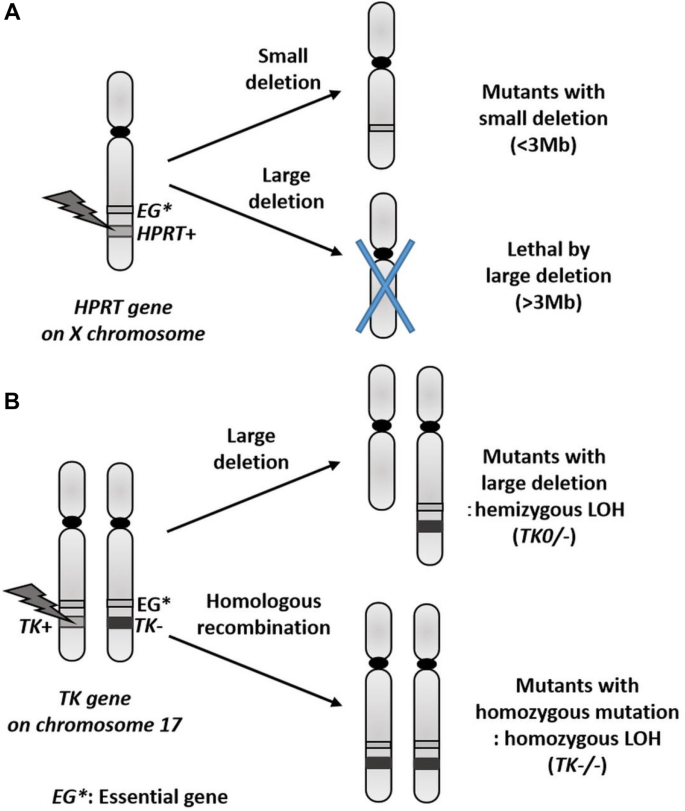
Possible mutations with deletion and LOH at the HPRT and the TK gene
Full size imageTK encodes thymidine kinase. Similar to HPRT, this gene may be considered as a marker in gene mutation testing, because a deficiency in the enzyme encoded by TK leads to the development of resistance of cells to trifluorothymidine. As TK is located on an autosome (chromosomes 17 and 11 in humans and mice, respectively), it should be heterozygous (TK+/−) for the detection of a recessive mutation. Therefore, this test can only be performed using specific cells. The mouse lymphoma cell line, L5178Y, and the human lymphoblastoid cell line, TK6, can be used for the TK mutation test, because an allele of TK in these cells harbours a point mutation generated by a chemical in advance. The testing system that uses L5178Y is termed the mouse lymphoma assay (MLA). The greatest advantage of the TK mutation test lies in its ability to be used to detect not only relatively small deletions and insertions in the TK gene, but also considerable deletions at the chromosomal level and recombination mutations between homologous chromosomes. The effect of the latter mutation is similar to that observed with the loss of heterozygosity (LOH), a change often detected in tumour suppressor genes during human tumourigenesis (Fig. 1b). Therefore, this testing system also demonstrates high significance as a model for the mechanism of cancer development. Thus, the TK mutation test can be used to detect a broad spectrum of mutations ranging from point mutations to considerable genetic changes at the chromosomal level. TK mutants possess two types of mutant cells that exhibit different proliferative capacities. The mutant cells with slower rates of proliferation exhibit substantial genetic changes at the chromosomal level. Therefore, classification of mutant cells affected by a particular chemical allows us to determine whether it is a mutagen that induces point mutations or a clastogen that induces chromosomal aberrations. OECD TG490 is a guideline for the TK mutation tests, i.e. MLA and TK6 gene mutation assay (OECD, 2015). However, the L5178Y cells that are used in MLA are genetically unstable and lead to the achievement of a high false-positive rate because these p53 mutant cells undergo abnormal apoptosis and present with abnormal cell cycle checkpoint mechanisms. Thus, TK6 is considered superior for use in gene mutation testing (Honma & Hayashi, 2011).
Chromosome aberration tests using mammalian cells (TG473)
Chromosome aberration testing, a technique used to explore structural and numerical (polyploidy) aberrations of chromosomes via optical microscopy, involves the subjection of cultured mammalian cells to treatment with a test substance and the preparation of chromosome specimens of cells in metaphase after a set period. This test enables the detection of transient chromosomal aberrations, which differ from stable chromosomal aberrations observed in hereditary diseases and/or cancer tissues. Thus, many cells that exhibit transient chromosomal aberration tend to die. Therefore, in a strict sense, this test does not help evaluate mutagenicity. However, chromosome aberration testing using mammalian cells is considered to be essential due to its utility in the detection of the direct genetic effects exerted by chemicals on mammalian cells that regulate complicated functions, which cannot be observed in bacterial cells which lack a chromosomal structure. Therefore, these are included in multiple test guidelines (Sofuni, 2005). Chinese hamster cell lines, CHO, CHL, and V79, which harbour a few large chromosomes and show a relatively stable karyotype, are widely used in this test due to the ease of handling and analysis. Additionally, primary human lymphocytes, such as the human lymphoblastoid cell line TK6, are also used.
Chromosomal aberrations are divided into numerical aberrations and structural aberrations. There are two basic types of structural aberrations. Chromosome-type aberrations involve an identical site in both chromatids of a chromosome. In contrast, chromatid-type aberrations involve only one chromatid of a chromosome. Each aberration can be further classified into two types. The first is a simple break-type aberration, while the second is an exchange-type aberration generated by mutual conversion at two or more break sites. Although exchange-type aberrations vary considerably, the basic mechanism is the same for both chromosome- and chromatid-type aberrations. A substance that causes structural chromosomal aberrations is referred to as a clastogen. Numerical aberrations consist of aneuploidy and polyploidy. The former refers to an increase or decrease in the number of chromosomes by one to several times, resulting in hyperdiploidy or hypodiploidy. In contrast, polyploidy is a phenomenon whereby the number of sets of a chromosome is multiplied to generate triploidy, tetraploidy, and so on. These result from the impairment of the division mechanism and/or cell fusion. The former is attributed to various factors, including the inhibition of cytoplasmic division, inhibition of cell division due to dysfunction of the spindle fibres, asymmetrical division due to non-disjunction of part of the chromosomes, and inhibition of the transition to mitotic phase or G2 phase.
Micronucleus test using mammalian cells (TG487)
The micronucleus test continues to gain increasing importance as a simple cytogenetic technique, because the analysis of chromosomes in the metaphase is time-consuming and the observation of chromosomes requires technical expertise. The micronucleus is a corpuscle composed of chromatin that exhibits staining in a similar manner as the main nucleus and contains a chromosomal fragment or a whole chromosome separate from the main nucleus. A chromosomal fragment or a chromosome that is not bound by spindle fibres is produced due to an abnormality in the chromosomal mitotic apparatus, which induces a structural or numerical chromosomal aberration. This fragment or whole chromosome does not migrate to the poles at the time of cell division, and as a result it is not incorporated into the main nucleus, thus remaining instead as a micronucleus. Thus, the production of a micronucleus indicates the occurrence of structural or numerical chromosomal aberrations. The in vitro micronucleus test involves examination of cells on slides prepared after the subjection of cells to treatment with a test substance, to detect the induction of chromosomal aberrations by conducting observations of interphase cells containing micronuclei in their cytoplasm. This simple test, considered to be equivalent to chromosome aberration testing, is used to detect aneugens as well as micronuclei containing a whole chromosome (Matsushima et al., 1999). The fluorescence in situ hybridisation (FISH) technique is used to differentiate between structural aberrations and aneuploidy, via the use of a DNA probe specific to the DNA sequence of the centromere of the chromosome. This confirms the presence or absence of the centromere in the micronucleus, allowing us to determine whether the micronucleus is a chromosomal fragment arising from a structural aberration or a whole chromosome generated by aneuploidy (Kirsch-Volders et al., 2003).
Cultured mammalian cells used for chromosome aberration testing can be used in this test. Cells that contain several chromosomes, a condition which renders observation difficult, may also be used easily. As the production of a micronucleus is dependent on cell division, a technique that involves the use of an actin polymerisation inhibitor, namely cytochalasin B (Cyto B), has been developed to identify dividing cells. In the presence of Cyto B, the nucleus of a cell divides to form a binuclear cell, allowing observation of only the micronucleus in the dividing cell. However, Cyto B is not used in cell lines that exhibit rapid proliferation because of their high cell division capacity.
In vivo tests
Erythrocyte micronucleus test (TG474)
The mammalian erythrocyte micronucleus test is an in vivo test that is conducted to measure the potency of chemicals that induce chromosomal aberrations; the test involves the enumeration of the number of immature (polychromatic) erythrocytes containing micronuclei in animal bone marrow or peripheral blood. Rodents are usually included for this test; however, the possibility of using other animal species (dogs, primates, and humans) has also been investigated. When a bone marrow erythroblast undergoes differentiation into an immature erythrocyte (also known as a polychromatic erythrocyte or reticulocyte) and moves into peripheral blood, the cell assumes an anucleate characteristic due to enucleation of the main nucleus. However, any micronucleus formed therein remains in the cytoplasm. At this stage, these cells lack a main nucleus, enabling comparatively easy visualisation and detection of their micronuclei. As micronuclei are generated from an acentric chromosome, late-segregating chromosome fragment, or a whole chromosome in certain cases, this test enables the detection of substances that induce chromosomal aberrations as well as substances that induce aneuploidy. The test substance is usually administered to the animals orally to mimic the exposure route in humans. Where bone marrow sample is used for the micronucleus test, specimens are usually prepared for micronucleus observation 18–24 h after the chemical has been administered once per day for 2 days (Hayashi, 1991).
In a manner similar to that adopted for the in vitro micronucleus test, FISH may be used to distinguish between substances that induce chromosomal aberrations (where a micronucleus that lacks a centromere is formed) and substances that induce aneuploidy (where a micronucleus with a centromere is formed). A positive micronucleus test result provides strong evidence and indicates that the substance exerts a high level of in vivo genotoxicity. However, because micronucleus formation is also induced by factors that do not cause DNA damage, such as a low body temperature and the enhancement of hematopoietic function, the result must be interpreted with caution. A negative micronucleus test indicates that the substance may not demonstrate any in vivo genotoxicity. However, in such a case, it is important to prove that the bone marrow has been sufficiently exposed to the test compound in a toxicokinetic manner or should demonstrate that a reduction in the proportion of immature erythrocytes has occurred.
In the erythrocyte micronucleus test, observations are automated using a flow cytometer or a laser scanning cytometer, partly due to the simplicity of observing the subject (Dertinger et al., 2011; Styles et al., 2001), Micronucleus test methods that target organs rather than erythrocytes have also been developed, rendering it possible to target many tissues, including the liver and digestive organs (Hamada et al., 2015).
Gene mutation tests using transgenic animals (TG488)
This test mainly uses transgenic mice and rats, such as MutaTMMouse, BigBlue®, and gpt delta (Nohmi et al., 2000; Thybaud et al., 2003). Multiple copies of a reporter gene are transferred to the genomes of these animals using λ phage DNA as the vector to facilitate the detection of mutations. The reporter gene is derived from a λ phage or a bacterium and is considered genetically neutral because it is not expressed in the animals. Therefore, there is no selection pressure due to its genetic function. Usually, the animals are exposed to a chemical, following which DNA derived from various organs (e.g. the liver, stomach, large intestine, and bone marrow) undergoes in vitro packaging into phage particles. The collected λ phages are then transferred to their host E. coli, enabling the detection of any mutation that has occurred in vivo in the animals, because such mutations are reflected in mutant E. coli. The greatest advantage of this test is that it may be used to detect a mutation in any organ, including germ cells, and may help ascertain whether the test substance induces cancer through its genotoxicity, via observation of the mutation in the carcinogenesis-afflicted target organ.
The test substance is usually administered to the animals via the oral route, but other methods of administration are possible. As mutations accumulate with each dose, multiple treatments are usually required before the ability of a substance to induce mutations can be detected. Where conventional tissues are used, it is recommended that the substance be administered once per day for 28 consecutive days, with sample collection on day 3 following the final administration. Additionally, from the perspective of animal ethics, it is recommended that this test be integrated with a general toxicity test, or be used in combination with another genotoxicity test, such as the micronucleus test, to reduce the number of animals that are used (Hori et al., 2019).
Comet assay (TG489)
The comet assay is used to detect initial DNA damage caused by a chemical (JaCVAM, 2009; Tice et al., 2000). The comet assay includes the following two options: the neutral assay and the alkaline assay. The neutral comet assay helps detect double-strand breaks in the DNA, whereas the alkaline comet assay helps detect not only double-strand breaks but also single-strand breaks and damaged DNA that contains an AP site (abasic site) and/or an alkali-sensitive site. The alkaline comet assay is more commonly used for genotoxicity testing. This assay may be performed using samples of any organ or tissue from which cells can be collected and isolated. The frequency and the route of administration of substances are similar to those of the micronucleus test. However, because this system aids the detection of initial damage, specimens must be prepared before any damage caused by the compound is repaired. Therefore, it is usually recommended that the test substance is administered two to three times and the specimens are prepared 2–6 h after the final administration.
To prepare specimens for the comet assay, a suspension of cells isolated from a part of an organ is gently mixed with a low-melting point agarose solution and mounted on glass slides. Following this, the specimens are immersed in an alkaline solution containing a detergent and a high concentration of salt for lysis of the plasma membrane and the nuclear membrane, and they are subjected to electrophoresis under highly alkaline conditions (pH > 13). The highly alkaline electrophoresis buffer causes double-strand DNA to unwind and dissociate into single strands, allowing electrophoretic detection of single-strand DNA breaks. As the migration rate of DNA during electrophoresis depends on the molecular weight, low-molecular-weight DNA fragments resulting from damage form a tail in the image, resembling a comet, the length of which is proportional to the damage level. To evaluate the comet assay result, an image analyser is used to quantitatively measure the potency of the substance for inducing DNA damage. The percentage of the DNA that is found in the tail (% tail DNA), the tail length, along with other factors, are used as indicators. Although the comet assay is generally considered highly sensitive, it is difficult to detect large DNA adducts using this assay. Additionally, apoptosis, a process which causes secondary DNA damage, frequently leads to the achievement of false-positive results. Therefore, the evaluation should be conducted carefully.
Pig-a assay (TG is under development)
The Pig-a (phosphatidylinositol glycan anchor biosynthesis, class A) assay is a recently developed in vivo gene mutation assay. Validation studies for the establishment of its OECD test guideline are ongoing (Miura et al., 2008). Glycosylphosphatidylinositol (GPI) anchors tether > 150 unique proteins to the outer surface of mammalian cells. Pig-a and additional 30 genes are involved in the biosynthesis of GPI anchors. As shown in Fig. 2, Pig-a is located on the X chromosome as one functional copy per cell, while all other genes are present in two copies.
Fig. 2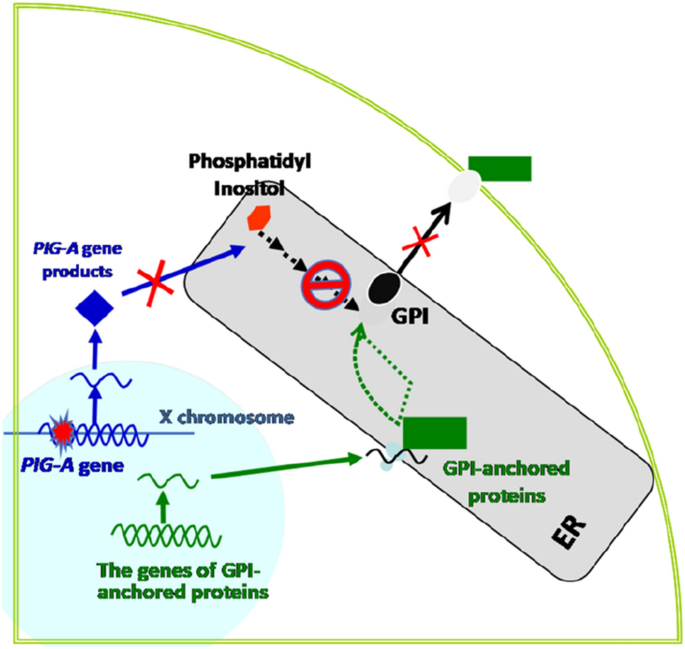
PIG-A assay
Full size imageTherefore, inactivating mutations in Pig-a result in a loss of GPI anchors as well as proteins tethered to the cell surface by GPI. It is commonly used in an in vivo gene mutation assay in rodents, wherein erythrocytes derived from small samples of peripheral blood are used to analyse the loss of GPI-anchored membrane proteins using flow cytometry, enabling assessment of the mutation frequency (MF) of the gene. The Pig-a assay is conducted using a small volume of peripheral blood obtained from the tail vein of animals, is more efficient and faster than rodent transgenic mutation tests, is easily integrated into general toxicological tests, and can be combined with other genotoxicity tests, thereby reducing the number of experiments required. The Pig-a in vivo gene mutation assay database is a useful resource that contains results of Pig-a gene mutation assays conducted in rats and mice. It is freely available from the following site: https://www.pharmacy.umaryland.edu/centers/cersi-files/.
The mutants measured in the erythrocyte Pig-a assay are formed in nucleated erythroid progenitor cells that are mainly located in the bone marrow, which implies that the test substance or its metabolites possess the ability to reach the bone marrow, thereby aiding the evaluation of adequate mutagenicity. This requirement is also necessary for the commonly used erythrocyte MN test. Pig-a MFs are more related to carcinogenicity predictions than the MN test or the comet assay, and show better sensitivity due to the accumulation of damage, as reported in a Tg.RasH2 mice carcinogenicity study (Chen et al., 2020). Compared with MN test and comet assay end points, which reflect acute genetic damage, Pig-a assay continuously reflects DNA damage over a relatively long period, because mutation induced by strong mutagens on stem/precursor cells can be amplified by clonal expansion with erythropoiesis. This assay is quick and inexpensive. However, the shortcoming is that mutated DNA is already gone in erythrocytes. Therefore, DNA sequencing or spectrum analysis of the mutations is impossible. Pig-a/PIG-A is reportedly highly conserved among species, and recent studies have mulled the possibility of extending the rodent Pig-a assay to a human erythrocyte PIG-A assay to investigate chemotherapy or radiation regimens in cancer patients or to assess populations occupationally exposed to genotoxicants (Cao, et al., 2020a, 2020b; Cao, et al., 2020a, 2020b; Haboubi et al., 2019). These studies declared PIG-A MFs as a potential, novel biomarker that could be used for monitoring genotoxicity in humans.
Testing using germ cells (TG478, 483, 485)
Testing techniques that involve the use of germ cells include the dominant lethal test using rodents, the mouse heritable translocation assay, and the chromosome aberration test using mammalian spermatogonia.
The dominant lethal test using rodents helps detect a genotoxicant that causes embryonic or foetal death via a dominant lethal mutation in the germ cells of a parent animal (usually a male rat or mouse) exposed to the substance. A dominant lethal mutation is generally caused by a structural or numerical chromosomal aberration, but may also be caused by a gene mutation and/or other toxic actions. The mouse heritable translocation test aids in the observation of structural chromosomal changes, mainly mutual translocations that develop in first-generation male mice exposed to a substance. Both tests are rarely used because of the considerable number of animals required. Currently, these tests are only performed by a few specialists.
Evaluation and interpretation of genotoxicity test results
In vitro test systems that involve the use of mammalian cells often yield false-positive results for carcinogenicity prediction in rodents (Kirkland et al., 2005). Though the cause of such false-positive results is often unknown, caution must be exercised because strong cytotoxicity, high osmotic pressure, precipitation, and non-physiological pH, among others, may lead to the achievement of a positive result via nonspecific effects (Scott & Roberts, 1987). Guidelines that provide information regarding the highest concentrations of the substance and the cytotoxicity levels that should be considered during testing must be rendered available to prevent the achievement of such false-positive results.
Positive in vitro results may indicate whether a chemical exhibits genotoxic characteristics. However, the biological significance of such positive in vitro results must be verified via accurate follow-up tests in many cases. Positive in vitro test results using mammalian cells are subjected to follow-up by selecting an appropriate in vivo test. Generally, an in vivo test with the same end point is selected. Additionally, as outlined in the guidelines pertaining to the pharmaceuticals mentioned previously, when a micronucleus test using a hematopoietic tissue has already been conducted, it is recommended that testing should be performed using a different tissue specimen. In contrast, in the case of the Ames test, a wide range of additional tests for the evaluation of mutagenicity and/or carcinogenicity may be necessary because a positive result for this test indicates that the test substance is reactive with DNA. Thus, in vitro gene mutation testing (HPRT/TK) using cultured mammalian cells, in vivo transgenic gene mutation testing, or the Pig-a assay, is recommended as a test for mutagenicity.
In cases where no follow-up test is available, or where the test substance also shows a positive reaction in the follow-up test (with evaluation as a genotoxicant or mutagen), it is important to properly assess the carcinogenic risk of the chemical.
Genotoxicity and carcinogenic risk assessment/management
As genotoxicity testing usually involves the qualitative test to detect the presence or absence of genotoxicity and/or mutagenicity, the result is considered either “positive” or “negative.” Such qualitative results of genotoxicity tests are considered for health risk assessments. In several cases, toxic chemical health risk assessments involve the use of a dose–response model based on theoretical and empirical studies, which include a threshold below which no health effects are detected. This allows the prescription of an acceptable daily intake (ADI). However, determination of an ADI poses challenges when a chemical has been found to be both carcinogenic and genotoxic. Unlike other toxicities, genotoxicity is not based on thresholds. Therefore, no ADI can be set because, theoretically, the health risk does not become zero unless intake becomes zero. This helps specify risk assessments for genotoxic carcinogens.
Mutagens are a type of genotoxicant that exhibit reactions with DNA to eventually cause gene mutation. Mutation rates never reach a value of zero because mutations are stochastic events. Additionally, even a single mutation in a gene that is important for cellular oncogenesis, such as an oncogene or a tumour suppressor gene, leads to the development of an original cancer cell, which may be sufficient to cause carcinogenesis. Therefore, the probability of oncogenesis cannot be completely eliminated either. Consequently, it is valid to consider that no threshold can be set for mutagens that cause irreversible mutations. However, a few researchers have suggested that a threshold is applicable to such mutagens. This concept is based on the contention that the induction of a mutation can be reduced to virtually zero because biological defence mechanisms, such as DNA repair, metabolic reactions, and scavengers, function efficiently at an extremely low dose range. However, this concept cannot be applied to risk assessment, because a technique demonstrating utilisation in the evaluation of such biological defence mechanisms, with applicability to test results, has not been developed.
Carcinogens are currently classified into two groups, i.e. mutagenic carcinogens and non-mutagenic (or sometimes called non-genotoxic) carcinogens. The target of the former is DNA while that of the latter is non-DNA such as proteins. It is generally accepted in regulatory sciences that there is no threshold for the risk of mutagenic carcinogens because even one base change in DNA may lead to cancer. In contrast, damage in protein does not necessarily accelerate carcinogenesis because there are multiple copies of the protein in the cell. Therefore, it is theoretically possible to set a threshold for non-mutagenic carcinogens. CA and MN tests detect both mutagenic and non-mutagenic carcinogens while Ames test detects only DNA-interacting mutagenic chemicals. The ICH (2014) established a Guideline on Assessment and Control of DNA Reactive (Mutagenic) Impurities in Pharmaceuticals to Limit Potential Carcinogenic Risk; M7 stated "the focus of this guideline is on DNA reactive substances that have a potential to directly cause DNA damage when present at low levels leading to mutations and therefore, potentially causing cancer (ICH, 2014). This type of mutagenic carcinogen is usually detected in a bacterial mutation (mutagenicity) assay. Other types of genotoxicants that are non-mutagenic typically have threshold mechanisms and usually do not pose carcinogenic risk in humans at the level ordinarily present as impurities". This principle is likely to exert a substantial impact on future risk assessments of these substances because it is applicable to chemicals and other substances that are present at trace levels in food, such as additives and residual agricultural chemicals.
Compared to the determination of a threshold that is associated with zero risk, an alternate method has been proposed for the assessment/management of the risk posed by genotoxicants, which is based on the concept that “even if a substance exhibits mutagenicity and/or genotoxicity, it may be considered virtually safe as long as the exposure dose is sufficiently low to result in extremely low carcinogenicity with a socially acceptable level of risk.” This exposure dose is termed a virtually safe dose (VSD) and is considered with an acceptable risk level of 10−5–10−6 (Honma, 2009) (Fig. 3).
Fig. 3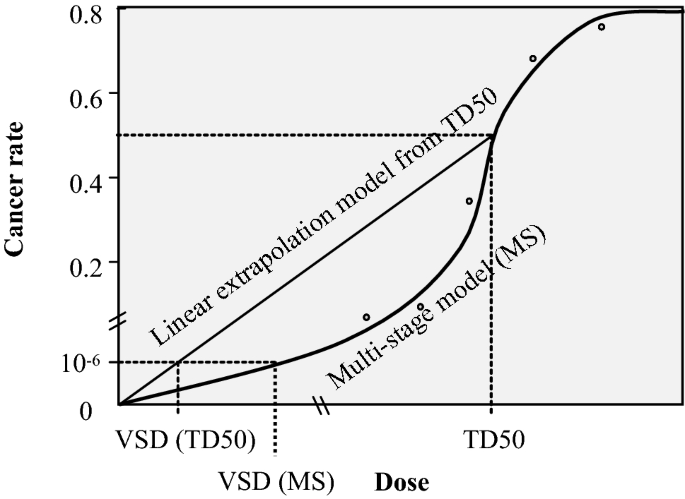
Calculation of virtually safe dose
Full size imageBased on carcinogen databases, it is possible to conclude that a majority of chemicals may pose only a minor virtual health hazard, even if they are mutagenic carcinogens, as long as their daily intake is below 1.5 μg/person/day. Such a comprehensive threshold is known as the “threshold of toxicological concern (TTC).” The use of the TTC approach has been established to regulate chemicals in several areas, such as food contact materials, food flavouring agents, and pharmaceutical impurities. The US FDA already uses the TTC for the risk management of chemicals eluted from plastic containers (indirect additives). Additionally, the Joint FAO/WHO Expert Committee on Food Additives (JECFA) has applied the TTC to additives that are used as flavouring agents in foods. In Japan, its application is under active discussion by the Food Safety Commission of Japan. It is noteworthy highly potent DNA-reactive carcinogens, such as aflatoxin-like, azoxy- or N-nitroso-carcinogens, are outside of the application of the TTC approach. The conservative degree or strictness of policy may depend on the usage of the chemicals, provide health benefits or not, the above-stated ICH-M7 guideline has prescribed a TTC level of 1.5 μg/person/day for managing mutagenic impurities in pharmaceuticals. EFSA/WHO proposed a level ten times lower, i.e. 0.15 µg/person/day or 0.0025 µg/kg bw/day, than that proposed by ICH-M7 guideline. For the regulation of the total carcinogenic risk on humans would be to establish weighted allocations for each class of chemicals, then, the total carcinogenic risk would be less than 1 × 10−5, even when people are exposed to multiple genotoxic carcinogens (Nohmi, 2018). Practical risk management methods, such as the relaxation of TTC levels for a pharmaceutical(s) during a medication period, have also been adopted, because the period of medication intake (medication period) is limited, compared with that of meals (over a lifetime), considering the low carcinogenicity of the compound based on its chemical structure information (compound-specific TTC) (ICH, 2014).
Advances in genotoxicity studies and testing
Cells used in genotoxicity tests
Most genotoxicity tests are conducted in in vitro systems, for which selection of appropriate cell lines is based on certain cell characteristics, including metabolic activity, and p53 status and origin (rodent versus human), among others. Bioactivation is necessary to induce genotoxicity in promutagens, but most cultured mammalian cells lack metabolic activity that initiates bioactivation. Although S9 rat liver fractions prepared from homogenised livers of rats have been commonly used for genotoxicity assessment, whether S9 completely simulates human metabolic conditions remains unclear owing to differences between these two species. For example, 2-acetaminofluorene, bio-activated using human S9 liver fractions, could not be activated using the rat S9-based metabolic activation system (Johnson et al., 1996). Although primary human or rodent hepatocytes seem to be promising, availability is limited. Human hepatocellular carcinoma cell lines, such as HepG2 and HepaRG, are other alternatives that can be used for predicting genotoxicity. HepG2 shows markedly low metabolic activity, while the HepRG cell line, which possesses better phase I and II metabolising potential, has been reported to be promising via genetic testing and is thus considered as a substitute that may be used to overcome the need of additional S9 (Gerets et al., 2012). Recent reports indicate that human-induced hepatocytes (hiHeps) (Liu et al., 2019), generated from fibroblasts via lentivirus-induced expression of liver transcription factors, express hepatic gene programmes, are amenable to expansion in vitro, and display functional characteristic of mature hepatocytes, thereby showing potential for application as an effective tool for in vitro genotoxic assessments. However, these warrant further validation. Meanwhile, human embryonic stem cells and induced pluripotent stem cell (iPSCs)-derived hepatocytes are considered a better choice for assessing safety in humans (Chapin & Stedman, 2009; Kang & Trosko, 2011). Since the use of embryonic stem cells involves ethical issues, the iPSCs, which rely on direct lineage reprogramming to induce the conversion of one specialised cell type into another lineage, allow the generation of intermediate pluripotent states as well as the avoidance of ethical issues associated with the use of human embryonic stem cells (Yamanaka & Blau, 2010).
Use of three-dimensional (3D) tissue equivalents in toxicology has been increasing over the last decade (Pfuhler et al., 2020). 3D liver, airway and skin-based models have been used in genotoxicity assays, among which 3D skin model-based genotoxicity assays have been used in the safety evaluations of cosmetic ingredients through dermal exposure starting from 10 years ago. Recently published literature reported that the 3D reconstructed human skin micronucleus (RSMN) assay was considered sufficiently validated through testing 43 coded chemicals in four US and European laboratories, and could be used as an animal-free alternative and also as a follow-up test for substances positive in standard in vitro genotoxicity assays (Pfuhler et al., 2021). The 3D spheroid format, which is readily constructed from hepatocytes, has been applied in the lymphocyte cytokinesis-block micronucleus (CBMN) assay, comet assay or -H2AX phosphorylation. HepG2/C3A cells cultured for 7 days in agarose-coated microplates formed spheroids and exhibited better sensitivity than 2D monolayers to DNA damage-induced compounds, as assessed by the comet assay and -H2AX phosphorylation (Coltman et al., 2021). Efforts are being made for applications of 3D models, including how to sufficiently cover the three key end points of genotoxicity, for example, human in vitro organotypic airway cultures were used as a model to assess DNA damage with the CometChip, and evaluate mutagenesis with Duplex Sequencing, respectively. (Wang et al., 2021).
Human lymphoblastoid TK6 cell line, a cell line of human origin expressing the wild-type p53 protein, was originally developed for conducting the TK gene mutation assay. TK6 cells can be handled easily and exhibit a high degree of efficiency for cell proliferation and colony formation, along with karyotype stability. These properties enable the application of TK6 in other genotoxicity assays, such as chromosome aberration tests, micronucleus tests, comet assays, and GreenScreen HC assays. Using genome editing technologies, such as CRISPR/Cas9, TALEN, and ZFN, various mutant TK6 cells that harbour genes regulating DNA repair, replication, recombination, and other processes associated with genome integrity have been developed (Honma et al., 2007; Keka et al., 2015; Takashima et al., 2009) Thus far, a consortium led by the National Institute of Health Sciences (NIHS) of Japan has reported the generation of nearly 135 types of TK6 mutant cells by implementing knock-out or knock-in of specific genes, and data are available from the NIHS homepage (http://www.nihs.go.jp/dgm/tk6.html). Some TK6 mutant cells display characteristic phenotypic behaviours, showing sensitivity or tolerance to a specific mutagen, as a result of which molecular and cytogenetic analyses can be performed to characterise the mutational spectrum and repair mechanism of specific mutagenic agents. Thus, TK6 cells show considerable potential applicability not only as a useful tool in genotoxicity evaluation, but also as an important tool in basic research pertaining to the investigation of DNA repair, mutagenesis, and genomic stability.
(Quantitative) structure–activity relationship ((Q)SAR)
Currently, over 100,000 industrial chemicals are present in our living environment. Not a few such chemicals may exert adverse effects on human health. Considering the rapid expansion in the number of industrial chemicals, international organizations and regulatory authorities have expressed the need for establishing effective screening tools to promptly and accurately identify chemical substances with potential adverse effects without conducting actual toxicological studies. Utilisation of (Q)SAR is a promising approach that aids the prediction of the potential adverse effects of a chemical based on its chemical structure. Significant efforts have been engaged in the development of (Q)SAR models that can help predict Ames mutagenicity, among other toxicological endpoints, mainly due to the significant amount of necessary Ames test data that have already been accumulated.
The International Council for Harmonization of Technical Requirements for Pharmaceuticals for Human Use (ICH) M7 guideline for the assessment and control of mutagenic impurities in pharmaceuticals was established in 2014. This is the first international guideline that addresses the use of (Q)SAR, instead of actual toxicological studies, for human health assessment. According to the guidelines, two complementary QSAR methodologies (statistics-based and expert rule-based) should be used to evaluate the chemical's potential to cause bacterial mutagenicity (Fig. 4).
Fig. 4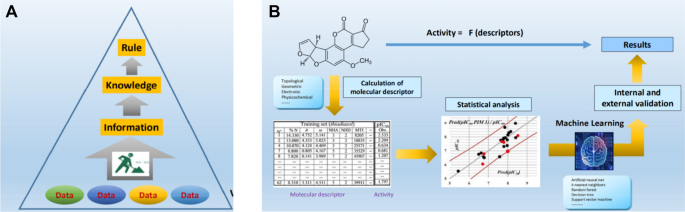
A Rule-based QSAR and B statistical-based QSAR (Honma, 2020)
Full size imageThe rule-based QSAR is a method in which characteristic substructures that give positive results are defined based on already known data, and Ames test results are predicted qualitatively using an established empirical rule. On the other hand, the statistics-based QSAR is based on physicochemical properties expressed as molecular descriptors (numerical data) such as geometric, electronic, and physicochemical descriptors, and descriptors that correlate highly with positive results in the Ames test, and is used to predict test results using machine learning methods.
An Ames/QSAR International Challenge Project with 12 QSAR vendors has been conducted by the NIHS of Japan, which aimed to validate and improve QSAR tools for predicting Ames mutagenicity of chemical substances. More than 12,000 chemicals have been evaluated during the first stage from 2014 to 2017, and stage 2 is currently ongoing. All QSAR tools demonstrated improved predictive power compared to the original versions. In future, an integrated approach for assessing the mutagenicity of chemical substances, in which both Ames tests and QSAR are integrated to deduce a final conclusion, may be used as a supporting tool for assessing actual test results (Honma, 2020).
Representative genotoxicity high-throughput in vitro assays
Traditional genotoxicity assessment techniques are not deemed suitable for high-throughput screening, owing to the tediousness of operational procedures, and thus more efficient and cost-effective methods are warranted for rapid evaluation of the potential genotoxicity of numerous chemical compounds. The Toxicology in the 21st Century (Tox21) program includes genotoxicity as one of the toxicological end points of interest to regulators and researchers (Mahadevan et al. 2011). Genotoxic substances may activate DNA damage responses in cells and exert effects on a series of characteristic genes and proteins involved in DNA damage response pathways, such as p53 and γH2AX, among others, leading to the generation of specific cellular responses, such as apoptosis, cell cycle arrest, and DNA repair (Bieging et al., 2014). Cell lines characterised by these markers are promising tools for genotoxicity assessment.
DNA repair-deficient DT40 cell lines for qHTS platform
Isogenic chicken DT40 B-lymphocyte cell lines, which are deficient in DNA repair pathways, may be used to identify genotoxins. These cell lines are considered a useful tool for quantitative high-throughput screening (qHTS) of genotoxic chemicals, as these cells are markedly sensitive to induction of DNA damage. DT40 cell lines are derived from a chicken B-lymphocyte line and are deemed suitable for studies related to DNA repair pathways associated with a short cell cycle, lack of a G1/S checkpoint, and a high percentage of cells in the S phase (Baba et al., 1985; Takao et al., 1999) With the qHTS platform, cells are automatically seeded and subjected to treatments in 1536-well plates using a commercial reagent dispenser, following which differential cytotoxicity is determined using a plate reader, which enables rapid screening of a substantial number of potential genotoxic compounds and provides more information regarding underlying mechanisms (Nishihara et al., 2016; Yamamoto et al., 2011).
The CellSensor p53RE-bla HCT-116 cell
The CellSensor p53RE-bla HCT-116 cell, developed by Invitrogen, which uses a beta-lactamase reporter gene under the control of p53 response elements stably integrated into human colon carcinoma HCT-116 cells, is used to indicate the effects of DNA damage-induced cytotoxicity. Detection of the fluorescence intensity of p53 response elements in HCT-116 cells enabled the screening of Tox21 compound library in a 1536-well plate format. Knight et al. used the HTS genotoxicity assay to analyse 320 predominantly pesticide-active compounds from the ToxCast™ research project, and the results demonstrated a high degree of efficiency and specificity (> 88%) compared with those of mutagenicity (Ames) and animal tumourigenicity data (Knight et al., 2009). However, HTS assays lack capacity for metabolic activation; therefore, HTS assays may be used for rapid prediction of numerous chemicals and may help provide supporting evidence for traditional genotoxicity experiments.
Automated analysis of DNA damage in the high-throughput version of the comet assay
Traditional comet assays involve manual handling of cells and the use of agarose film, staining, focussing, imaging, and scoring procedures, all of which are labour intensive and time consuming and result in an extremely low-throughput performance (Bajpayee et al., 2019). To address the above-mentioned limitations, researchers have adopted the following two approaches: high throughput and automation of the entire procedure. Originally, users developed a high-throughput version of the comet assay based on the use of a multi-chamber plate (MCP) (Stang & Witte, 2009, 2010; Witte et al., 2007) This specially designed 96-well plate helps avoid individual processing of each sample and allows the electrophoresis of cellular DNA from 96 samples in the same run. Micropatterned comet assay has been considered as another strategy for high throughput. In 2016, Hanna et al. developed a mini-gel version of the comet assay (eight gels per slide), which proved to be time efficient and yielded extensive information on DNA strand breaks, oxidative DNA damage, and photogenotoxicity (Di Bucchianico et al., 2017). Microfabrication techniques further enable the production of micrometre-scale arrays of cells on one slide. The development of CometChip enables the preparation of multiple samples in parallel on the same assay substrate and facilitates integration with custom or commercially available software for detection and scoring of comets, thereby improving efficiency and reducing human bias, as compared to the standard comet assays (Ge et al., 2015; Tay et al., 2020; Watson et al., 2014). Automation includes pre-treatment of cell–agarose suspension and image analysis. Unlike the commonly used semi-manual analysis software, OpenComet (Gyori et al., 2014), CometQ (Ganapathy et al., 2016) and HiComet (Lee et al., 2018) are reported to be fully automated analytical tools that can be used for recognition and quantification of different types of comet assay images, which accelerates the rate-limiting step and requires less intervention. Additionally, the integration of automated liquid workstation further reduces manual manipulation and improves efficiency. However, high investment in the purchase of costly equipment needs consideration (Albert et al., 2016).
Automated imaging and counting of γH2AX foci
H2AX, a member of the H2A family of chromosomal histones, is subjected to rapid phosphorylation to γH2AX in response to the induction of DNA double-strand breaks (DSBs). Within minutes of DNA damage, γH2AX gathers at the damage site and forms γH2AX foci to initiate the repair of DSBs (Mah et al., 2010). Since the level of γ-H2AX evidently reflects the degree of DNA damage, it is considered to be an important molecular marker of DNA damage, which may be used to predict the genotoxicity of environmental chemicals and compounds.
Since the initial development of two-dimensional gel electrophoresis for γH2AX detection (Rogakou et al., 1998), more sensitive and visible methods have been developed based on γH2AX-specific fluorophore-labelled antibodies. Analysis of γH2AX via microscopy is a laborious and highly subjective procedure, owing to the need for manual operation (Sedelnikova et al., 2002; Zhou et al., 2006) and poses difficulties in the improvement of throughput. Flow cytometry provides a possible resolution to the need for rapid quantification of γH2AX in considerable cell populations. It automatically detects the fluorescence of γH2AX recognised by antibodies and displays a degree of efficiency and sensitivity comparable to that observed with other in vitro genotoxicity assays (Bryce et al., 2018; Smart et al., 2011) However, samples are tested only once and are destroyed following detection, and thus cannot be used in periodic, dynamic analysis of γ-H2AX. High-content screening (HCS) is a newly developed approach for automated dynamic imaging and analysis of substantial numbers of experimental data points such as foci number and distribution (Ando et al., 2014; Hopp et al., 2017) This method, which yields quantitative information on γH2AX based on high-speed confocal image acquisition and built-in analysis software, is a promising technique that may enhance the efficacy of high-throughput genotoxicity screening assays.
Automated scoring of the cytokinesis-block micronucleus cytome assay
The CBMN assay, which was originally developed for the measurement of MN in binucleated (BN) cells, recently has evolved into a comprehensive cytome method to include biomarkers that measure chromosomal instability and mitotic activity by quantification of nuclear buds (NBUDs), nucleoplasmic bridges (NPBs) and enumeration of mono- and polynucleated cells (Rodrigues et al., 2018). There has been significant progresses towards automation of the CBMN assay to eliminate individual bias and to enhance sample throughput and statistical power. Several software systems have been developed, e.g. Metafer™ slide-scoring module (Metasystems, Germany) (Rossnerova et al., 2011) and PathFinder™ Cellscan™ system (IMSTAR, Paris, France) (Decordier et al., 2011). Additionally, laser scanning cytometry systems have been developed, validated and used to visualise MN as well as the cytoplasms of BN cells (François et al., 2014). Recently, the CBMN assay has been adapted to imaging flow cytometry (IFC), which allows direct visualisation of the cytoplasm using brightfield (BF) imagery together with the main nuclei and MN using fluorescent imagery (Wang et al., 2019). By using IFC, images from cells in suspensions can be captured, allowing visualisation of intact cytoplasmic membranes, and DNA content, mono-, bi- and polynucleated cells with and without MN can be rapidly and automatically identified and quantified.
High-dimensional/high information content assays for genetic toxicology testing
Presently, high-dimensional, high-content data sources are considered to facilitate increases in throughput and mechanistic information in genotoxicity testing. Experimental approaches presented include adductomics, cellular phenotype-based assays, global transcriptional profiling, and error-reduced single-molecule sequencing based on next-generation sequencing (NGS).
Mass spectrometry-based DNA adductomics
Covalent binding between many genotoxicants and DNA, following metabolic activation by intrinsic enzymes, leads to the formation of DNA adducts that provide the most direct molecular evidence for evaluating DNA damage (Luch, 2005). Thus, the detection of DNA adducts serves as a biomarker of environmental exposure and facilitates an understanding of the molecular mechanism of disease (Liu et al., 2017).
Liquid chromatography–tandem mass spectrometry (LC–MS/MS) is a promising platform for characterisation and quantitation of DNA adducts. Owing to advances in MS, DNA adductomics has emerged as a powerful tool for comprehensive detection of both targeted and untargeted DNA adducts as well as for screening of total DNA damage in the human genome (Guo & Turesky, 2019). In an MS/MS analysis, 2'-deoxynucleosides and their adducts showed a characteristic fragment, which indicated the neutral loss of the 2'-deoxyribose moiety (Chan et al., 2007). This feature serves as a high-throughput scanning strategy termed “pseudo-constant neutral loss” (P-CNL) in DNA adductomic studies (Kanaly et al., 2006; Villalta & Balbo, 2017). Figure 5 illustrates the determination of the formation of DNA adducts induced by AA IVa, an aristolochic acid analogue. The reaction mixture of AA IVa with dA was examined by using LC–MS/MS under the neutral loss mode with the mass loss of 116, that is, the loss of deoxyribose moiety (Fig. 5).
Fig. 5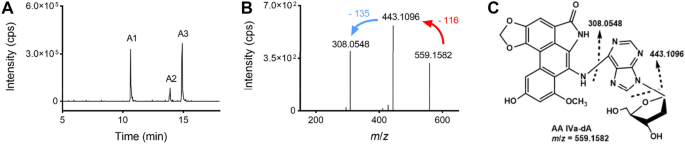
A typical LC–MS/MS chromatogram of the reaction mixture of AA IVa with dA under the neutral loss mode (the mass loss was set at 116). To identify the adducts, the chromatogram obtained was extracted with the m/z value of the expected adducts and the result was presented. A The chromatogram extracted with m/z 559, which is the m/z value for AA IVa-dA adducts. B The product ion mass spectrum of A1 in Fig. 5A shows the fragmentation of the protonated molecular ion. C The tentative structure of an AA IVa-dA adduct as supported by the HRMS measurement
Full size imageTriple quadrupole-MS (QqQ-MS) is the preferred method for detecting putative DNA adducts in samples with high sensitivity and selectivity. It allows quantitative detection of these DNA adducts and aids tentative identification by aiding the obtainment of fragment spectra via generation of product ion scanning patterns. For example, Lambert et al. utilised QqQ-MS via neutral loss scanning to analyse multiple putative DNA adducts from lung tissues of non-smokers and smokers, and to generate the adductomics map (Lambert et al., 2015). Another study reported the screening of 12 DNA adducts in calf thymus DNA (in a mixture of formaldehyde) as potential biomarkers (Chang et al., 2021). Ion trap-MS (IT-MS) is used to perform multistage MS (MSn) scanning to obtain more structural information in addition to the function of QqQ, a feature which improves the detection specificity (Luch, 2005). For example, IT-MS enabled the quantitative identification of aristolochic acid I/II (AA I/II)-induced DNA adducts in rodents as well as in the renal cortex DNA of patients with upper urinary tract (Yun et al., 2012). Another study reported the utilisation of IT-MS to identify and quantify acylfulvene/illudin S–DNA adducts in colon cancer cell lines (Pietsch et al., 2013). High-resolution accurate mass spectrometry (HRAM) has been considered to perform screening for multiple DNA adducts (Ishino et al., 2015). HRAM is used to acquire spectral data with precise mass to determine the molecular formula of an ion. Further fragmentation data were obtained via MS2 or MS3 analyses to confirm chemical structures, thus highlighting the advantages associated with qualitative analyses when investigating unknown DNA adducts. For example, 90 DNA adducts were identified in vitro using the adductomics-HRAM analysis, of which 12 showed potential applicability as genotoxic markers that could be used to predict colorectal cancer risks associated with the consumption of red meat (Hemeryck et al., 2018).
DNA adductomics is a developing technology. With the availability of powerful analytical methods, DNA adducts databases, and data processing tools, DNA adductomics technologies are expected to enable the detection of human samples, leading to a better understanding of the interaction between chemical exposures and cancer risk. Investigation of the association between DNA adducts and mutations will provide insights into new pathways for elucidating the chemical causes of cancer and for understanding cancer development mechanisms.
High-content cellular phenotype-based assays
High-content phenotype-based screening assays involve the use of parameters in addition to conventional genetic end points to obtain complementary information regarding genotoxic mechanisms, to help achieve high-throughput capabilities dependent on the utilisation of flow cytometric or image analysis-based technologies. The in vitro ToxTracker®, a commercial platform, is a mouse embryonic stem reporter cell-based flow cytometric assay (Hendriks et al., 2016). It combines information derived from six different GFP reporter cell lines relevant to DNA damage (Bscl 2 and Rtkn genes), oxidative stress (Srxn 1 and Blvrb genes), protein unfolding (Ddit 3 gene), and general cellular stress (Btg2 gene). Relative to individual genotoxicity assays, the ToxTracker method demonstrates an advantage of being used in the identification of differences between toxic potencies of compounds in a single assay. This system has been substantially validated, and an initiative has been launched to develop an OECD guideline for it. Additionally, the in vitro Multiflow assay is a high-throughput genotoxic assay that multiplexes several biomarkers, including γH2AX (indicator of DNA double-strand breaks), p53 (indicator of p53 activation to DNA damage), phospho-histone H3 (detection of mitotic cells), and polyploidisation, into a single flow cytometry-based assay (Wilde et al., 2017). Compared to the traditional, standard genotoxicity assays, Multiflow is a rapid and effective method that helps identify genotoxic hazards, distinguishes between clastogenic and aneugenic action, and provides information on molecular targets and potency. This system has been validated widely across multiple laboratories, and artificial intelligence is now being applied to improve its predictive power. Finally, the Multi-Endpoint MEGA system is based on a confocal microscopy image analysis and machine learning ensemble (Winkelbeiner et al., 2020). Several end points are distinguished via confocal microscopy using a test which involves specific staining of different cell structures. These staining procedures are as follows: (i) kinetochore labelling to detect aneugenicity and clastogenicity; (ii) γH2AX to identify clastogenicity; and (iii) Hoechst staining to analyse the cell cycle. Currently, verification results indicate that this method is highly sensitive and specific.
Global transcriptional profiling by NGS
NGS is a powerful platform that revolutionises both DNA and RNA analyses. RNA-seq, the principal NGS platform for transcriptome analysis, has been applied in biomarker discovery. For example, the Toxicogenomics/JEMS study group for transcriptome analysis has reported its application in biomarker discovery and has reported the distinguishing between genotoxic and non-genotoxic hepatocarcinogens in rodent liver (Furihata et al., 2018); the discovery highlighted genes associated with the Trp53-mediated signalling pathway for DNA damage response. Another long non-coding RNA (lncRNA) study reported that 25 new lncRNAs that were differentially expressed in the livers of rats subchronically exposed to aflatoxin B1 (AFB1) showed potential applicability as predictive biomarkers of hepatocellular carcinoma (Merrick et al., 2018). These studies demonstrated the ability to derive transcriptomic signatures predictive of in vivo genotoxic and non-genotoxic effects in a variety of different models and showed the utility of such signatures in cancer risk assessment.
In vitro screening using toxicogenomic biomarkers has been proposed as a first-tier screen in assessments of chemicals. Li et al. developed and validated an in vitro transcriptomic biomarker responsive to DNA damage-inducing agents in human cells (Li et al., 2015, 2017), namely TGx-DDI, for genotoxicity testing. They recently reported that the TGx-DDI Plexset assay could assess genotoxicity in a simple and rapid way (Chen et al., 2021), enabling multiplexed gene expression assays to be performed more efficiently and cost effectively for projects ranging up to 96 RNA targets. The assay could also provide additional mechanistic information to augment current genotoxicity hazard assessment and thus was proposed to be widely used in genotoxicity screening.
Error-reduced NGS
Several selection-based assays have been performed for mutagenicity evaluation due to the stochastic and infrequent nature of mutagenesis. Most such assays rely on reporter genes and only assess mutations indirectly based on phenotype, and this relies on the use of specific experimental models as well as sophisticated experimental operations, leading to bias generation in mutation detection. Recently, NGS technologies have presented potential, new techniques for genomic exploration, demonstrating the feasibility and applicability of the tools with reduced costs and faster turnaround times. However, conventional NGS methods are associated with high error rates (10–2–10–3) that obscure true mutations which occur less frequently than errors (Shendure & Ji, 2008), because the spontaneous mutation rate in normal somatic mammalian cells is estimated to be in the range of 10–8–10–9 mutations per nucleotide per cell division (Lynch, 2010). Several approaches have been considered to improve the accuracy of NGS-based detection of environmental mutagen-induced mutations, where most approaches may be grouped into three main strategies as follows: (i) computational and statistical strategies to exclude sequences of low confidence (Wei et al., 2011; Wilm et al., 2012); (ii) library preparation protocols aimed at removing mutagenically damaged nucleotides in templates, such as ultrasonic shearing of DNA into short fragments, production of energy that breaks the phosphodiester backbone, reduction of oxidising bases that leads to artefactual C:G A:T transition mutations by regulating pH buffer (Costello et al., 2013), and removal of 8-oxo-dG and cytidine deamination, two of the most common DNA damaging events (Nishimura, 2011), via the action of damage-specific glycosylases FPG and UDG, respectively (Arbeithuber et al., 2016); and (iii) molecule consensus sequencing, which is considered as the most successful strategy and has been described in subsequent sections (Kennedy et al., 2014; Schmitt et al., 2012) This strategy highlights the possibility of directly determining genome-wide ultra-rare mutations of any organism.
The principal underlying consensus sequencing strategy is to obtain redundant copies arising from similar templates, usually via PCR amplification for error correction. As shown in Fig. 6, true mutations are expected to be present in all or in the majority of these multiple copies, and any variant that occurs only in a portion of the homogeneous copies is considered as a sequencing artefact (Fig. 6) (You, 2021).
Fig. 6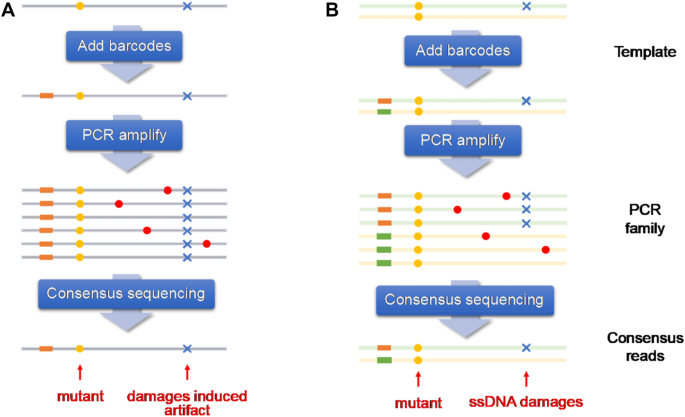
A Single-strand-based consensus sequencing and B Duplex strand-based consensus sequencing (You, 2021)
Full size imageThis strategy also requires molecular barcodes for each original DNA fragment that is propagated to all daughter molecules during amplification and sequencing. The duplex sequencing technique, developed by groups from the University of Washington (Salk et al., 2018), uses a special form of molecular tagging to independently barcode each strand of individual DNA duplexes, sequencing copies of both strands of a DNA, to remove sequencing errors in individual reads and PCR errors in copies of one of the two strands (Schmitt et al., 2012). Duplex sequencing presents with a theoretical error rate of less than 10−9 errors per base pair (bp), which is lower than the typical mutational load of human tissues, and therefore raises the possibility of quantifying somatic mutation rates in genetically heterogeneous samples, via the detection of somatic mutations in single DNA molecules.
Generally, PCR amplification is necessary to obtain sufficient copies for consensus sequencing. Therefore, the raw sequencing depth required for consensus sequencing ought to be markedly higher to obtain yields that are similar to those obtained by using conventional NGS methods. This results in an exponential increase in sequencing costs, especially when such approaches are applied to genome-wide mutation screening. Reportedly, several attempts have been engaged to overcome the above-mentioned issue. Bottleneck sequencing system (BotSeqS) (Hoang et al., 2016), which combines molecular barcoding used in a simple dilution step immediately prior to library amplification, has successfully reduced the sequencing depth, but the efficiency remains markedly low. The most recently reported method, Nanorate sequencing (Nanoseq), based on duplex sequencing and BotSeqS, was developed by scientists at the Sanger Institute. Nanoseq reduces error rates to less than five errors per billion calls, which is markedly lower than that observed in typical somatic mutation rates. This is achieved by limiting errors during the preparation of DNA libraries. Nanoseq involves the use of blunt-end restriction enzymes to fragment DNA and dideoxy bases (ddBTPs) to avoid nick extension (Abascal et al., 2021). A mathematically modelled dilution step is also performed to optimise the efficiency and yield of the method. ‘Hawk-Seq™’, a short-read platform, utilises dsDNA consensus sequences by determining the optimal input DNA amount for PCR to regulate the overlap rate of DNA fragments, to construct libraries, to achieve maximum sequence efficiency, and to cover large genomic regions (Matsumura et al., 2019). These were used to analyse mutagen-induced genomic mutations in gpt delta mice and Salmonella typhimurium TA100 exposed to various mutagens, and generated accurate (c.a. 1 error/107–108 bp) (Otsubo et al., 2021), high-resolution mutational profiles that helped identify characteristic features of each mutagen. Paired-end and complementary consensus sequencing (PECC-Seq) uses a simple modified PCR-free sequencing workflow and a repair enzyme to avoid extension of internal nicks and error-prone end repair, to reduce the error rate of NGS in mutation detection from 10–3 ~ 10–2 to lower than 5/10–8, which is comparable to the spontaneous mutation frequencies of mammalian genomes (10–9 ~ 10–7), and thereby may increase the consensus-making efficiency and may be applicable on a whole genome scale (You et al., 2020).
Third-generation NGS, such as PacBio SMRT and Oxford Nanopore, also show potential in error-reduced sequencing (Clark et al., 2011). An advantage of single-molecule sequencing platforms is that many DNA adducts can be directly detected without prior manipulation. In the case of the PacBio SMRT sequencing technology, chemical modifications to the template base affect the kinetics of dNTP incorporation by DNA polymerases in a defined manner that is relatively specific to each adduct (Revollo et al., 2021).
Error-reduced NGS, which enables genome-wide analysis of low-frequency mutations in non-cancer cells, may be utilised as a tool to reveal the effects of various environmental mutagens and may also help understand the pathophysiology of many diseases including cancer. Firstly, for the purpose of providing information pertaining to regulatory decisions, the error-reduced NGS shows potential applicability as a substitute for traditional in vivo mutagenesis tests and could be used in the evaluation of the mutagenicity of a chemical via the estimation of mutation burdens on the genome and identification of the mutational signatures. It may be integrated into general toxicity evaluation tests since no special animal model is needed. Secondly, it may be applied to basic mechanism studies of carcinogenesis to characterise the acquisition and evolution of mutational spectra during carcinogenesis and to detect the relatively rare mutations that occur early following mutagen exposure. The technique may finally dissect the mutational complexity, which might be the consequence of accompanying DNA damage repair, oxidative damage, inflammation, etc., and reveal the degree of clonality of later stage tumours that arise from the carcinogenic process. Thirdly, an increase in the mutation burdens of normal cells derived from invasive samples of high-risk populations may be considered an effective biomarker. The biomarker can be combined with mutational spectra and exposure biomarkers, e.g. internal exposure levels or DNA adduct formation, and used in cancer risk prediction. Fourthly, the technique can be applied to the risk assessments of complicated environmental factors, such as smoking, food, and outdoor air pollution. The identified mutational signatures of a specific chemical on any target organ in animal experiments can be compared with the data of the COSMIC (see https://cancer.sanger.ac.uk/signatures/) or TCGA database (see https://www.cancer.gov/tcga), and then the information is used collectively to clarify individual contributions of complicated environmental chemicals to the associated human cancer risks. Finally, it may be useful in the life sciences for the issues that remain to be clarified, such as mutation and ageing and the origin of de novo mutations. It can also be used to evaluate the safety of emerging techniques, including stem cell therapy and gene editing. Taken together, error-reduced NGS, combined with other rapidly developing omics study tools, may play an increasingly important role in mutagenicity research.
References
Abascal, F., Harvey, L. M. R., Mitchell, E., Lawson, A. R. J., Lensing, S. V., Ellis, P., & Martincorena, I. (2021). Somatic mutation landscapes at single-molecule resolution. Nature, 593(7859), 405–410. https://doi.org/10.1038/s41586-021-03477-4
Albert, O., Reintsch, W. E., Chan, P., & Robaire, B. (2016). HT-COMET: A novel automated approach for high throughput assessment of human sperm chromatin quality. Human Reproduction, 31(5), 938–946. https://doi.org/10.1093/humrep/dew030
Ando, M., Yoshikawa, K., Iwase, Y., & Ishiura, S. (2014). Usefulness of monitoring γ-H2AX and cell cycle arrest in HepG2 cells for estimating genotoxicity using a high-content analysis system. Journal of Biomolecular Screening, 19(9), 1246–1254. https://doi.org/10.1177/1087057114541147
Arbeithuber, B., Makova, K. D., & Tiemann-Boege, I. (2016). Artifactual mutations resulting from DNA lesions limit detection levels in ultrasensitive sequencing applications. DNA Research, 23(6), 547–559. https://doi.org/10.1093/dnares/dsw038
Baba, T. W., Giroir, B. P., & Humphries, E. H. (1985). Cell lines derived from avian lymphomas exhibit two distinct phenotypes. Virology, 144(1), 139–151. https://doi.org/10.1016/0042-6822(85)90312-5
Bajpayee, M., Kumar, A., & Dhawan, A. (2019). The comet assay: assessment of in vitro and in vivo DNA damage. Methods in Molecular Biology, 2031, 237–257. https://doi.org/10.1007/978-1-4939-9646-9_12
Bieging, K. T., Mello, S. S., & Attardi, L. D. (2014). Unravelling mechanisms of p53-mediated tumour suppression. Nature Reviews Cancer, 14(5), 359–370. https://doi.org/10.1038/nrc3711
Bryce, S. M., Bernacki, D. T., Smith-Roe, S. L., Witt, K. L., Bemis, J. C., & Dertinger, S. D. (2018). Investigating the generalizability of the MultiFlow ® DNA damage assay and several companion machine learning models with a set of 103 diverse test chemicals. Toxicological Sciences, 162(1), 146–166. https://doi.org/10.1093/toxsci/kfx235
Cao, Y., Wang, T., Xi, J., Zhang, G., Wang, T., Liu, W., & Luan, Y. (2020a). PIG-A gene mutation as a genotoxicity biomarker in human population studies: An investigation in lead-exposed workers. Environmental and Molecular Mutagenesis, 61(6), 611–621. https://doi.org/10.1002/em.22373
Cao, Y., Wang, X., Liu, W., Feng, N., Xi, J., You, X., & Luan, Y. (2020b). The potential application of human PIG-A assay on azathioprine-treated inflammatory bowel disease patients. Environmental and Molecular Mutagenesis, 61(4), 456–464. https://doi.org/10.1002/em.22348
Chan, W., Zheng, Y., & Cai, Z. (2007). Liquid chromatography-tandem mass spectrometry analysis of the DNA adducts of aristolochic acids. Journal of the American Society for Mass Spectrometry, 18(4), 642–650. https://doi.org/10.1016/j.jasms.2006.11.010
Chang, Y. J., Cooke, M. S., Chen, Y. R., Yang, S. F., Li, P. S., Hu, C. W., & Chao, M. R. (2021). Is high resolution a strict requirement for mass spectrometry-based cellular DNA adductomics? Chemosphere, 274, 129991. https://doi.org/10.1016/j.chemosphere.2021.129991
Chapin, R. E., & Stedman, D. B. (2009). Endless possibilities: Stem cells and the vision for toxicology testing in the 21st century. Toxicological Sciences, 112(1), 17–22. https://doi.org/10.1093/toxsci/kfp202
Chen, R., Lin, Y. T., Fornace, A. J., Jr., & Li, H. H. (2021). A high-throughput and highly automated genotoxicity screening assay. Altex. https://doi.org/10.14573/altex.2102121
Chen, R., Zhou, C., Cao, Y., Xi, J., Ohira, T., He, L., & Luan, Y. (2020). Assessment of Pig-a, micronucleus, and comet assay endpoints in Tg.RasH2 mice carcinogenicity study of aristolochic acid I. Environmental and Molecular Mutagenesis, 61(2), 266–275. https://doi.org/10.1002/em.22325
Clark, T. A., Spittle, K. E., Turner, S. W., & Korlach, J. (2011). Direct detection and sequencing of damaged DNA bases. Genome Integr, 2, 10. https://doi.org/10.1186/2041-9414-2-10
Coltman, N. J., Coke, B. A., Chatzi, K., Shepherd, E. L., Lalor, P. F., Schulz-Utermoehl, T., & Hodges, N. J. (2021). Application of HepG2/C3A liver spheroids as a model system for genotoxicity studies. Toxicology Letters, 345, 34–45. https://doi.org/10.1016/j.toxlet.2021.04.004
Costello, M., Pugh, T. J., Fennell, T. J., Stewart, C., Lichtenstein, L., Meldrim, J. C., & Getz, G. (2013). Discovery and characterization of artifactual mutations in deep coverage targeted capture sequencing data due to oxidative DNA damage during sample preparation. Nucleic Acids Research, 41(6), e67. https://doi.org/10.1093/nar/gks1443
Decordier, I., Papine, A., Vande Loock, K., Plas, G., Soussaline, F., & Kirsch-Volders, M. (2011). Automated image analysis of micronuclei by IMSTAR for biomonitoring. Mutagenesis, 26(1), 163–168. https://doi.org/10.1093/mutage/geq063
Dertinger, S. D., Torous, D. K., Hayashi, M., & MacGregor, J. T. (2011). Flow cytometric scoring of micronucleated erythrocytes: An efficient platform for assessing in vivo cytogenetic damage. Mutagenesis, 26(1), 139–145. https://doi.org/10.1093/mutage/geq055
Di Bucchianico, S., Cappellini, F., Le Bihanic, F., Zhang, Y., Dreij, K., & Karlsson, H. L. (2017). Genotoxicity of TiO2 nanoparticles assessed by mini-gel comet assay and micronucleus scoring with flow cytometry. Mutagenesis, 32(1), 127–137. https://doi.org/10.1093/mutage/gew030
François, M., Hochstenbach, K., Leifert, W., & Fenech, M. F. (2014). Automation of the cytokinesis-block micronucleus cytome assay by laser scanning cytometry and its potential application in radiation biodosimetry. BioTechniques, 57(6), 309–312. https://doi.org/10.2144/000114239
Furihata, C., Toyoda, T., Ogawa, K., & Suzuki, T. (2018). Using RNA-Seq with 11 marker genes to evaluate 1,4-dioxane compared with typical genotoxic and non-genotoxic rat hepatocarcinogens. Mutation Research, Genetic Toxicology and Environmental Mutagenesis, 834, 51–55. https://doi.org/10.1016/j.mrgentox.2018.07.002
Ganapathy, S., Muraleedharan, A., Sathidevi, P. S., Chand, P., & Rajkumar, R. P. (2016). CometQ: An automated tool for the detection and quantification of DNA damage using comet assay image analysis. Computer Methods and Programs in Biomedicine, 133, 143–154. https://doi.org/10.1016/j.cmpb.2016.05.020
Ge, J., Chow, D. N., Fessler, J. L., Weingeist, D. M., Wood, D. K., & Engelward, B. P. (2015). Micropatterned comet assay enables high throughput and sensitive DNA damage quantification. Mutagenesis, 30(1), 11–19. https://doi.org/10.1093/mutage/geu063
Gerets, H. H., Tilmant, K., Gerin, B., Chanteux, H., Depelchin, B. O., Dhalluin, S., & Atienzar, F. A. (2012). Characterization of primary human hepatocytes, HepG2 cells, and HepaRG cells at the mRNA level and CYP activity in response to inducers and their predictivity for the detection of human hepatotoxins. Cell Biology and Toxicology, 28(2), 69–87. https://doi.org/10.1007/s10565-011-9208-4
Guo, J., & Turesky, R. J. (2019). Emerging technologies in mass spectrometry-based DNA adductomics. High Throughput. https://doi.org/10.3390/ht8020013
Gyori, B. M., Venkatachalam, G., Thiagarajan, P. S., Hsu, D., & Clement, M. V. (2014). OpenComet: An automated tool for comet assay image analysis. Redox Biology, 2, 457–465. https://doi.org/10.1016/j.redox.2013.12.020
Haboubi, H. N., Lawrence, R. L., Rees, B., Williams, L., Manson, J. M., Al-Mossawi, N., & Jenkins, G. J. (2019). Developing a blood-based gene mutation assay as a novel biomarker for oesophageal adenocarcinoma. Scientific reports, 9(1), 5168. https://doi.org/10.1038/s41598-019-41490-w
Hamada, S., Ohyama, W., Takashima, R., Shimada, K., Matsumoto, K., Kawakami, S., & Hayashi, M. (2015). Evaluation of the repeated-dose liver and gastrointestinal tract micronucleus assays with 22 chemicals using young adult rats: Summary of the collaborative study by the Collaborative Study Group for the Micronucleus Test (CSGMT)/The Japanese Environmental Mutagen Society (JEMS) - Mammalian Mutagenicity Study Group (MMS). Mutation Research, Genetic Toxicology and Environmental Mutagenesis, 780–781, 2–17. https://doi.org/10.1016/j.mrgentox.2015.01.001
Hayasi, M. (1991). 小核試験 : 実験法からデータの評価まで. サイエンティスト社. (林真). https://iss.ndl.go.jp/books/R100000002-I000002117592-00?ar=4e1f&locale=zh
Hemeryck, L. Y., Rombouts, C., De Paepe, E., & Vanhaecke, L. (2018). DNA adduct profiling of in vitro colonic meat digests to map red vs. white meat genotoxicity. Food and Chemical Toxicology, 115, 73–87. https://doi.org/10.1016/j.fct.2018.02.032
Hendriks, G., Derr, R. S., Misovic, B., Morolli, B., Calléja, F. M., & Vrieling, H. (2016). The extended toxtracker assay discriminates between induction of dna damage, oxidative stress, and protein misfolding. Toxicological Sciences, 150(1), 190–203. https://doi.org/10.1093/toxsci/kfv323
Hoang, M. L., Kinde, I., Tomasetti, C., McMahon, K. W., Rosenquist, T. A., Grollman, A. P., & Papadopoulos, N. (2016). Genome-wide quantification of rare somatic mutations in normal human tissues using massively parallel sequencing. Proceedings of National Academy of Science USA, 113(35), 9846–9851. https://doi.org/10.1073/pnas.1607794113
Honma, M. (2009). 遺伝毒性物質に閾値はあるのか?(話題). ファルマシア, 45(2), 143–148. https://doi.org/10.14894/faruawpsj.45.2_143 本間正充.
Honma, M. (2020). An assessment of mutagenicity of chemical substances by (quantitative) structure-activity relationship. Genes Environ, 42, 23. https://doi.org/10.1186/s41021-020-00163-1
Honma, M., & Hayashi, M. (2011). Comparison of in vitro micronucleus and gene mutation assay results for p53-competent versus p53-deficient human lymphoblastoid cells. Environmental and Molecular Mutagenesis, 52(5), 373–384. https://doi.org/10.1002/em.20634
Honma, M., Sakuraba, M., Koizumi, T., Takashima, Y., Sakamoto, H., & Hayashi, M. (2007). Non-homologous end-joining for repairing I-SceI-induced DNA double strand breaks in human cells. DNA Repair (amst), 6(6), 781–788. https://doi.org/10.1016/j.dnarep.2007.01.004
Hopp, N., Hagen, J., Aggeler, B., & Kalyuzhny, A. E. (2017). Automated high-content screening of γ-H2AX Expression in HeLa cells. Methods in Molecular Biology, 1554, 273–283. https://doi.org/10.1007/978-1-4939-6759-9_20
Hori, H., Shimoyoshi, S., Tanaka, Y., Momonami, A., Masumura, K., Yamada, M., Fujii, W., & Kitagawa, Y. (2019). Integration of micronucleus tests with a gene mutation assay in F344 gpt delta transgenic rats using benzo[a]pyrene. Mutation Research, Genetic Toxicology and Environmental Mutagenesis, 837, 1–7. https://doi.org/10.1016/j.mrgentox.2018.09.003
https://linkinghub.elsevier.com/retrieve/pii/S1383-5718(18)30049-4
ICH. (2011). S2(R1) guidance on genotoxicity testing and data interpretation for pharmaceuticals intended for human use. ICH.
ICH. (2014). M7, assessment and control of dna reactive (mutagenic) impurities in pharmaceuticals to limit potential carcinogenic risk. ICH.
Ishino, K., Kato, T., Kato, M., Shibata, T., Watanabe, M., Wakabayashi, K., & Totsuka, Y. (2015). Comprehensive DNA adduct analysis reveals pulmonary inflammatory response contributes to genotoxic action of magnetite nanoparticles. International Journal of Molecular Sciences, 16(2), 3474–3492. https://doi.org/10.3390/ijms16023474
JaCVAM. (2009). The protocol of an international validation study on the in vivo rodent alkaline Comet assay. http://cometassay.com/JaCVAM.pdf. Accessed 30 Nov 2009
Johnson, T. E., Umbenhauer, D. R., & Galloway, S. M. (1996). Human liver S-9 metabolic activation: Proficiency in cytogenetic assays and comparison with phenobarbital/beta-naphthoflavone or aroclor 1254 induced rat S-9. Environmental and Molecular Mutagenesis, 28(1), 51–59. https://doi.org/10.1002/(sici)1098-2280(1996)28:1%3c51::Aid-em8%3e3.0.Co;2-h
Kanaly, R. A., Hanaoka, T., Sugimura, H., Toda, H., Matsui, S., & Matsuda, T. (2006). Development of the adductome approach to detect DNA damage in humans. Antioxidants and Redox Signaling, 8(5–6), 993–1001. https://doi.org/10.1089/ars.2006.8.993
Kang, K.-S., & Trosko, J. E. (2011). Stem cells in toxicology: fundamental biology and practical considerations. Toxicological Sciences, 120(suppl_1), S269–S289. https://doi.org/10.1093/toxsci/kfq370
Keka, I. S., Mohiuddin, M. Y., Rahman, M. M., Sakuma, T., Honma, M., & Sasanuma, H. (2015). Smarcal1 promotes double-strand-break repair by nonhomologous end-joining. Nucleic Acids Research, 43(13), 6359–6372. https://doi.org/10.1093/nar/gkv621
Kennedy, S. R., Schmitt, M. W., Fox, E. J., Kohrn, B. F., Salk, J. J., Ahn, E. H., & Loeb, L. A. (2014). Detecting ultralow-frequency mutations by Duplex Sequencing. Nature Protocols, 9(11), 2586–2606. https://doi.org/10.1038/nprot.2014.170
Kirkland, D., Aardema, M., Henderson, L., & Müller, L. (2005). Evaluation of the ability of a battery of three in vitro genotoxicity tests to discriminate rodent carcinogens and non-carcinogens I. Sensitivity, specificity and relative predictivity. Mutation Research, 584(1–2), 1–256. https://doi.org/10.1016/j.mrgentox.2005.02.004
Kirsch-Volders, M., Sofuni, T., Aardema, M., Albertini, S., Eastmond, D., Fenech, M., & Wakata, A. (2003). Report from the in vitro micronucleus assay working group. Mutation Research, 540(2), 153–163. https://doi.org/10.1016/j.mrgentox.2003.07.005
Knight, A. W., Little, S., Houck, K., Dix, D., Judson, R., Richard, A., & Walmsley, R. M. (2009). Evaluation of high-throughput genotoxicity assays used in profiling the US EPA ToxCast chemicals. Regulatory Toxicology and Pharmacology, 55(2), 188–199. https://doi.org/10.1016/j.yrtph.2009.07.004
Lambert, M., Meudec, E., Verbaere, A., Mazerolles, G., Wirth, J., Masson, G., & Sommerer, N. (2015). A high-throughput UHPLC-QqQ-MS method for polyphenol profiling in rosé wines. Molecules, 20(5), 7890–7914. https://doi.org/10.3390/molecules20057890
Lee, T., Lee, S., Sim, W. Y., Jung, Y. M., Han, S., Won, J. H., & Yoon, S. (2018). HiComet: A high-throughput comet analysis tool for large-scale DNA damage assessment. BMC Bioinformatics, 19(Suppl 1), 44. https://doi.org/10.1186/s12859-018-2015-7
Li, H. H., Chen, R., Hyduke, D. R., Williams, A., Frötschl, R., Ellinger-Ziegelbauer, H., & Fornace, A. J., Jr. (2017). Development and validation of a high-throughput transcriptomic biomarker to address 21st century genetic toxicology needs. Proceedings of National Academy of Science USA, 114(51), E10881-e10889. https://doi.org/10.1073/pnas.1714109114
Li, H. H., Hyduke, D. R., Chen, R., Heard, P., Yauk, C. L., Aubrecht, J., & Fornace, A. J., Jr. (2015). Development of a toxicogenomics signature for genotoxicity using a dose-optimization and informatics strategy in human cells. Environmental and Molecular Mutagenesis, 56(6), 505–519. https://doi.org/10.1002/em.21941
Liu, B., van Gerwen, M., Bonassi, S., & Taioli, E. (2017). Epidemiology of environmental exposure and malignant mesothelioma. Journal of Thoracic Oncology, 12(7), 1031–1045. https://doi.org/10.1016/j.jtho.2017.04.002
Liu, W., Xi, J., Cao, Y., You, X., Chen, R., Zhang, X., & Luan, Y. (2019). An adaption of human-induced hepatocytes to in vitro genetic toxicity tests. Mutagenesis, 34(2), 165–171. https://doi.org/10.1093/mutage/gey041
Luch, A. (2005). Nature and nurture – lessons from chemical carcinogenesis. Nature Reviews Cancer, 5(2), 113–125. https://doi.org/10.1038/nrc1546
Lynch, M. (2010). Rate, molecular spectrum, and consequences of human mutation. Proceedings of the National Academy of Science USA, 107(3), 961–968. https://doi.org/10.1073/pnas.0912629107
Mah, L. J., El-Osta, A., & Karagiannis, T. C. (2010). gammaH2AX: A sensitive molecular marker of DNA damage and repair. Leukemia, 24(4), 679–686. https://doi.org/10.1038/leu.2010.6
Mahadevan, B., Snyder, R. D., Waters, M. D., Benz, R. D., Kemper, R. A., Tice, R. R., & Richard, A. M. (2011). Genetic toxicology in the 21st century: reflections and future directions. Environ Mol Mutagen 52(5):339–354. https://doi.org/10.1002/em.20653
Maron, D. M., & Ames, B. N. (1983). Revised methods for the Salmonella mutagenicity test. Mutation Research, 113(3–4), 173–215. https://doi.org/10.1016/0165-1161(83)90010-9
Matsumura, S., Sato, H., Otsubo, Y., Tasaki, J., Ikeda, N., & Morita, O. (2019). Genome-wide somatic mutation analysis via Hawk-Seq™ reveals mutation profiles associated with chemical mutagens. Archives of Toxicology, 93(9), 2689–2701. https://doi.org/10.1007/s00204-019-02541-3
Matsushima, T., Hayashi, M., Matsuoka, A., Ishidate, M., Miura, F. K., Shimizu, H., & Sofuni, H. (1999). Validation study of the in vitro micronucleus test in a Chinese hamster lung cell line (CHL/IU). Mutagenesis, 14(6), 569–580. https://doi.org/10.1093/mutage/14.6.569
Merrick, B. A., Chang, J. S., Phadke, D. P., Bostrom, M. A., Shah, R. R., Wang, X., & Wright, G. M. (2018). HAfTs are novel lncRNA transcripts from aflatoxin exposure. PLoS ONE, 13(1), e0190992. https://doi.org/10.1371/journal.pone.0190992
Miura, D., Dobrovolsky, V. N., Mittelstaedt, R. A., Kasahara, Y., Katsuura, Y., & Heflich, R. H. (2008). Development of an in vivo gene mutation assay using the endogenous Pig-A gene: II. Selection of Pig-A mutant rat spleen T-cells with proaerolysin and sequencing Pig-A cDNA from the mutants. Environmental and Molecular Mutagenesis, 49(8), 622–630. https://doi.org/10.1002/em.20413
Mortelmans, K., & Riccio, E. S. (2000). The bacterial tryptophan reverse mutation assay with Escherichia coli WP2. Mutation Research, 455(1–2), 61–69. https://doi.org/10.1016/s0027-5107(00)00076-2
Mortelmans, K., & Zeiger, E. (2000). The Ames Salmonella/microsome mutagenicity assay. Mutation Research, 455(1–2), 29–60. https://doi.org/10.1016/s0027-5107(00)00064-6
Nishihara, K., Huang, R., Zhao, J., Shahane, S. A., Witt, K. L., Smith-Roe, S. L., & Xia, M. (2016). Identification of genotoxic compounds using isogenic DNA repair deficient DT40 cell lines on a quantitative high throughput screening platform. Mutagenesis, 31(1), 69–81. https://doi.org/10.1093/mutage/gev055
Nishimura, S. (2011). 8-Hydroxyguanine: A base for discovery. DNA Repair (amst), 10(11), 1078–1083.
Nohmi, T. (2018). Thresholds of genotoxic and non-genotoxic carcinogens. Toxicological Research, 34(4), 281–290. https://doi.org/10.5487/TR.2018.34.4.281
Nohmi, T., Suzuki, T., & Masumura, K. (2000). Recent advances in the protocols of transgenic mouse mutation assays. Mutation Research, 455(1–2), 191–215. https://doi.org/10.1016/s0027-5107(00)00077-4
OECD. (2010). OECD guideline for testing of chemicals, full list of test guidelines. OECD.
OECD. (2015). Test no. 490, in vitro mammalian cell gene mutation tests using the thymidine kinase gene. OECD.
Otsubo, Y., Matsumura, S., Ikeda, N., & Morita, O. (2021). Hawk-Seq™ differentiates between various mutations in Salmonella typhimurium TA100 strain caused by exposure to Ames test-positive mutagens. Mutagenesis, 36(3), 245–254. https://doi.org/10.1093/mutage/geab006
Pfuhler, S., Downs, T. R., Hewitt, N. J., Hoffmann, S., Mun, G. C., Ouedraogo, G., & Aardema, M. J. (2021). Validation of the 3D reconstructed human skin micronucleus (RSMN) assay: An animal-free alternative for following-up positive results from standard in vitro genotoxicity assays. Mutagenesis, 36(1), 1–17. https://doi.org/10.1093/mutage/geaa035
Pfuhler, S., van Benthem, J., Curren, R., Doak, S. H., Dusinska, M., Hayashi, M., & Corvi, R. (2020). Use of in vitro 3D tissue models in genotoxicity testing: Strategic fit, validation status and way forward. Report of the working group from the 7(th) International Workshop on Genotoxicity Testing (IWGT). Mutation Research Genetic Toxicology and Environmental Mutagenesis, 850–851, 503135. https://doi.org/10.1016/j.mrgentox.2020.503135
Pietsch, K. E., van Midwoud, P. M., Villalta, P. W., & Sturla, S. J. (2013). Quantification of acylfulvene- and illudin S-DNA adducts in cells with variable bioactivation capacities. Chemical Research in Toxicology, 26(1), 146–155. https://doi.org/10.1021/tx300430r
Revollo, J. R., Miranda, J. A., & Dobrovolsky, V. N. (2021). PacBio sequencing detects genome-wide ultra-low-frequency substitution mutations resulting from exposure to chemical mutagens. Environmental and Molecular Mutagenesis, 62(8), 438–445. https://doi.org/10.1002/em.22462
Rodrigues, M. A., Beaton-Green, L. A., Wilkins, R. C., & Fenech, M. F. (2018). The potential for complete automated scoring of the cytokinesis block micronucleus cytome assay using imaging flow cytometry. Mutat Res Genet Toxicol Environ Mutagen, 836(Pt A), 53–64. https://doi.org/10.1016/j.mrgentox.2018.05.003https://europepmc.org/articles/PMC6435702?pdf=renderhttps://europepmc.org/articles/PMC6435702
Rogakou, E. P., Pilch, D. R., Orr, A. H., Ivanova, V. S., & Bonner, W. M. (1998). DNA double-stranded breaks induce histone H2AX phosphorylation on serine 139. Journal of Biological Chemistry, 273(10), 5858–5868. https://doi.org/10.1074/jbc.273.10.5858
Rossnerova, A., Spatova, M., Schunck, C., & Sram, R. J. (2011). Automated scoring of lymphocyte micronuclei by the metasystems metafer image cytometry system and its application in studies of human mutagen sensitivity and biodosimetry of genotoxin exposure. Mutagenesis, 26(1), 169–175. https://doi.org/10.1093/mutage/geq057
Salk, J. J., Schmitt, M. W., & Loeb, L. A. (2018). Enhancing the accuracy of next-generation sequencing for detecting rare and subclonal mutations. Nature Reviews Genetics, 19(5), 269–285. https://doi.org/10.1038/nrg.2017.117
Schmitt, M. W., Kennedy, S. R., Salk, J. J., Fox, E. J., Hiatt, J. B., & Loeb, L. A. (2012). Detection of ultra-rare mutations by next-generation sequencing. Proceedings of the National Academy of Science USA, 109(36), 14508–14513. https://doi.org/10.1073/pnas.1208715109
Scott, D., & Roberts, S. A. (1987). Extrapolation from in vitro tests to human risk: Experience with sodium fluoride clastogenicity. Mutation Research, 189(1), 47–58. https://doi.org/10.1016/0165-1218(87)90032-2
Sedelnikova, O. A., Rogakou, E. P., Panyutin, I. G., & Bonner, W. M. (2002). Quantitative detection of (125)IdU-induced DNA double-strand breaks with gamma-H2AX antibody. Radiation Research, 158(4), 486–492. https://doi.org/10.1002/1097-0320(20010601)44:2<153::AID-CYTO1095>3.0.CO;2-H
Shendure, J., & Ji, H. (2008). Next-generation DNA sequencing. Nature Biotechnology, 26(10), 1135–1145. https://doi.org/10.1038/nbt1486
Smart, D. J., Ahmedi, K. P., Harvey, J. S., & Lynch, A. M. (2011). Genotoxicity screening via the γH2AX by flow assay. Mutation Research, 715(1–2), 25–31. https://doi.org/10.1016/j.mrfmmm.2011.07.001
Sofuni, T. (2005). 染色体異常試験 センショクタイ イジョウ シケン. サイエンティスト社. (祖父尼, 俊雄). http://lib.kyoto-wu.ac.jp/opc/recordID/catalog.bib/BA73202779
Stang, A., & Witte, I. (2009). Performance of the comet assay in a high-throughput version. Mutation Research, 675(1–2), 5–10. https://doi.org/10.1016/j.mrgentox.2009.01.007
Stang, A., & Witte, I. (2010). The ability of the high-throughput comet assay to measure the sensitivity of five cell lines toward methyl methanesulfonate, hydrogen peroxide, and pentachlorophenol. Mutation Research, 701(2), 103–106. https://doi.org/10.1016/j.mrgentox.2010.04.011
Styles, J. A., Clark, H., Festing, M. F. W., & Rew, D. A. (2001). Automation of mouse micronucleus genotoxicity assay by laser scanning cytometry. Cytometry, 44(2), 153–155. https://doi.org/10.1002/1097-0320(20010601)44:2%3c153::AID-CYTO1095%3e3.0.CO;2-H
Takao, N., Kato, H., Mori, R., Morrison, C., Sonada, E., Sun, X., & Yamamoto, K. (1999). Disruption of ATM in p53-null cells causes multiple functional abnormalities in cellular response to ionizing radiation. Oncogene, 18(50), 7002–7009. https://doi.org/10.1038/sj.onc.1203172
Takashima, Y., Sakuraba, M., Koizumi, T., Sakamoto, H., Hayashi, M., & Honma, M. (2009). Dependence of DNA double strand break repair pathways on cell cycle phase in human lymphoblastoid cells. Environmental and Molecular Mutagenesis, 50(9), 815–822. https://doi.org/10.1002/em.20481
Tay, I. J., Park, J. J. H., Price, A. L., Engelward, B. P., & Floyd, S. R. (2020). HTS-compatible cometchip enables genetic screening for modulators of apoptosis and DNA double-strand break repair. SLAS Discovery, 25(8), 906–922. https://doi.org/10.1177/2472555220918367
Thybaud, V., Dean, S., Nohmi, T., de Boer, J., Douglas, G. R., Glickman, B. W., & Yajima, N. (2003). In vivo transgenic mutation assays. Mutation Research, 540(2), 141–151. https://doi.org/10.1016/j.mrgentox.2003.07.004
Tice, R. R., Agurell, E., Anderson, D., Burlinson, B., Hartmann, A., Kobayashi, H., & Sasaki, Y. F. (2000). Single cell gel/comet assay: Guidelines for in vitro and in vivo genetic toxicology testing. Environmental and Molecular Mutagenesis, 35(3), 206–221. https://doi.org/10.1002/(SICI)1098-2280(2000)35:3%3c206::AID-EM8%3e3.0.CO;2-J
Villalta, P. W., & Balbo, S. (2017). The future of DNA adductomic analysis. International Journal of Molecular Sciences. https://doi.org/10.3390/ijms18091870
Wang, Q., Rodrigues, M. A., Repin, M., Pampou, S., Beaton-Green, L. A., Perrier, J., & Wilkins, R. C. (2019). Automated triage radiation biodosimetry: Integrating imaging flow cytometry with high-throughput robotics to perform the cytokinesis-block micronucleus assay. Radiation Research, 191(4), 342–351. https://doi.org/10.1667/RR15243.1
Wang, Y., Mittelstaedt, R. A., Wynne, R., Chen, Y., Cao, X., Muskhelishvili, L., & Heflich, R. H. (2021). Genetic toxicity testing using human in vitro organotypic airway cultures: Assessing DNA damage with the CometChip and mutagenesis by Duplex Sequencing. Environmental and Molecular Mutagenesis, 62(5), 306–318. https://doi.org/10.1002/em.22444
Watson, C., Ge, J., Cohen, J., Pyrgiotakis, G., Engelward, B. P., & Demokritou, P. (2014). High-throughput screening platform for engineered nanoparticle-mediated genotoxicity using CometChip technology. ACS Nano, 8(3), 2118–2133. https://doi.org/10.1021/nn404871p
Wei, Z., Wang, W., Hu, P., Lyon, G. J., & Hakonarson, H. (2011). SNVer: A statistical tool for variant calling in analysis of pooled or individual next-generation sequencing data. Nucleic Acids Research, 39(19), e132. https://doi.org/10.1093/nar/gkr599
Wilde, S., Dambowsky, M., Hempt, C., Sutter, A., & Queisser, N. (2017). Classification of in vitro genotoxicants using a novel multiplexed biomarker assay compared to the flow cytometric micronucleus test. Environmental and Molecular Mutagenesis, 58(9), 662–677. https://doi.org/10.1002/em.22130
Wilm, A., Aw, P. P., Bertrand, D., Yeo, G. H., Ong, S. H., Wong, C. H., & Nagarajan, N. (2012). LoFreq: A sequence-quality aware, ultra-sensitive variant caller for uncovering cell-population heterogeneity from high-throughput sequencing datasets. Nucleic Acids Research, 40(22), 11189–11201. https://doi.org/10.1093/nar/gks918
Winkelbeiner, N., Wandt, V. K., Ebert, F., Lossow, K., Bankoglu, E. E., Martin, M., & Schwerdtle, T. (2020). A multi-endpoint approach to base excision repair incision activity augmented by parylation and DNA damage levels in mice: Impact of sex and age. International Journal of Molecular Sciences. https://doi.org/10.3390/ijms21186600
Witte, I., Plappert, U., de Wall, H., & Hartmann, A. (2007). Genetic toxicity assessment: Employing the best science for human safety evaluation part III: The comet assay as an alternative to in vitro clastogenicity tests for early drug candidate selection. Toxicological Sciences, 97(1), 21–26. https://doi.org/10.1093/toxsci/kfl192
Yamamoto, K. N., Hirota, K., Kono, K., Takeda, S., Sakamuru, S., Xia, M., & Tice, R. R. (2011). Characterization of environmental chemicals with potential for DNA damage using isogenic DNA repair-deficient chicken DT40 cell lines. Environmental and Molecular Mutagenesis, 52(7), 547–561. https://doi.org/10.1002/em.20656
Yamanaka, S., & Blau, H. M. (2010). Nuclear reprogramming to a pluripotent state by three approaches. Nature, 465(7299), 704–712. https://doi.org/10.1038/nature09229
You, X. (2021). Environmental agents-induced ultra-low frequency mutation detection by molecular consensus sequencing. Shanghai Jiao Tong University.
You, X., Thiruppathi, S., Liu, W., Cao, Y., Naito, M., Furihata, C., & Suzuki, T. (2020). Detection of genome-wide low-frequency mutations with paired-end and complementary consensus sequencing (PECC-Seq) revealed end-repair-derived artifacts as residual errors. Archives of Toxicology, 94(10), 3475–3485. https://doi.org/10.1007/s00204-020-02832-0
Yun, B. H., Rosenquist, T. A., Sidorenko, V., Iden, C. R., Chen, C. H., Pu, Y. S., & Turesky, R. J. (2012). Biomonitoring of aristolactam-DNA adducts in human tissues using ultra-performance liquid chromatography/ion-trap mass spectrometry. Chemical Research in Toxicology, 25(5), 1119–1131. https://doi.org/10.1021/tx3000889
Zhou, C., Li, Z., Diao, H., Yu, Y., Zhu, W., Dai, Y., & Yang, J. (2006). DNA damage evaluated by gammaH2AX foci formation by a selective group of chemical/physical stressors. Mutation Research, 604(1–2), 8–18. https://doi.org/10.1016/j.mrgentox.2005.12.004
Acknowledgements
This work is supported by the Major Program of the National Natural Science Foundation of China, grant number 41991314.
Funding
This work is supported by the Major Program of the National Natural Science Foundation of China, grant number 41991314.
Author information
Affiliations
Shanghai Jiao Tong University School of Medicine, 225 South Chongqing Rd., Shanghai, 200025, China
Yang Luan
National Institute of Health Sciences, 3-25-26 Tonomachi, Kawasaki-Ku, Kanagawa, 210-9501, Japan
Masamitsu Honma
Corresponding authors
Correspondence to Yang Luan or Masamitsu Honma.
Ethics declarations
Conflict of interest
There are no conflicts of interest to declare.
Rights and permissions
About this article
Cite this article
Luan, Y., Honma, M. Genotoxicity testing and recent advances. GENOME INSTAB. DIS. (2021). https://doi.org/10.1007/s42764-021-00058-7
Received19 October 2021
Revised22 November 2021
Accepted26 November 2021
Published04 December 2021
Share this article
Anyone you share the following link with will be able to read this content:
Get shareable linkKeywords
Genotoxicity testing
High dimensional
High information content
High-throughput assays
Next-generation sequencing (NGS)
用户登录
还没有账号?
立即注册