The role of ral signaling and post translational modifications (PTMs) of Ras in cancer
Review Article
Mohammad Reza Zinatizadeh, Peyman Kheirandish Zarandi, Mahsa Keshavarz-Fathi, Mohammad Hadi Yousefi & Nima Rezaei
Genome Instability & Disease , 3 22–32 (2022)
Abstract
Mutation in RAS gene is one of the most common genetic alterations, which seems to be seen in one third of human cancers. Ras, as a molecular switch, has been considered in wide variety of signaling pathways such as cell division and apoptosis. Ras proteins have a binary function to transmit different extracellular messages into intracellular signaling network. It has been proved that Ras proteins associate with different plasma membranes. Although all Ras isoforms have been found at plasma membrane, H-Ras and N-Ras are located in Golgi, and K-Ras at ER and mitochondria outer membrane. There have been a lot of efforts to inhibit Ras signaling that can be a pivotal approach to treat Ras-induced tumors. Effect of RalA and RalB on the growth of embryonal tumors, at downstream region of Ras, has been studied in a number of studies, which showed that inhibition of these signaling pathways can provide a strong therapeutic approach to cancer. Also, post translational modifications (PTMs) in proteins interfere extremely with cell signaling pathways in cells that can react to external signals. In this review, the role of Ral signaling in cancer and PTM of Ras proteins has been reviewed.
Introduction
More than a third of human cancers are induced by RAS mutations. RalA and RalB (Ras-like) are two small GTPases (20–25 kDa G-proteins), known as a Ras-related GTPases. The intrinsic GDP-GTP exchange of Ral proteins are activated by Ral guanine nucleotide exchange factors (RalGEFs), while the GTPase activity is increased by two GTPase activating proteins (RalGAPs), an important negative regulator of RalA/RalB (Fig. 1a) (Fruman & Rommel, 2014; Gentry, Martin, Reiner, & Der, 2014; Shirakawa & Horiuchi, 2015; Yan et al., 2014). RalA and RalB are localized at the plasma membrane. Moreover, they are associated with Golgi, endosome and mitochondria (Chen et al., 2006; Fernández et al., 2011; Shipitsin & Feig, 2004). The concept of Ral localization is important, it can provide more effective activation of Ral effectors only in special regions. C-terminal of Ral proteins include C = cysteine, A = aliphatic amino acids, and X = any amino acid (CAAX) motif that is modified as post translation to regulate localization in specific membranes (Falsetti et al., 2007; Michaelson et al., 2005). During protein maturation, the presence of leucine at X location lead to the addition of two geranylgeranyltransferase-I (GGTase-I) proteins, which catalyzes the transfer of the farnesyl and geranylgeranyl groups to proteins containing a C-terminal CAAX motif to the first cysteine, then via Ras converting enzyme (RCE1) AAX is cleaved. Finally, methylation of cysteine, modified by isoprenylcysteine carboxyl methyltransferase (ICMT), is performed (Gentry et al., 2015; Nishimura & Linder, 2013). Recent studies have demonstrated that RalA and RalB require RCE1 to target the plasma membrane (H. Wang, Hossain, et al., 2010; Wang, Owens, et al., 2010). Furthermore, serine phosphorylation of these proteins are transferred to various cytoplasmic membranes and induces ubiquitination (Table 1) (Lim et al., 2010; Neyraud et al., 2012). RalA phosphorylation stimulates GTP binding, while RalB ubiquitination induces its interaction with Sec5 and Exo84 regulating autophagy (Sablina et al., 2007; Simicek et al., 2013). Some of these protein modifications are important for tumor growth. Previous studies showed that inhibition of Ral phosphorylation stop tumor growth in pancreatic cancer (Martin et al., 2012). RalB is phosphorylated by protein kinase Cα (PKCα) and protein kinase A (PKA). As a result, it increases the rearrangement of the cytoskeleton and vesicle trafficking (Bivona et al., 2006). Inhibition of RalB phosphorylation can prevent tumor growth and metastasis, especially in bladder cancer (McLaughlin & Aderem, 1995). It is well stablished that Ral plays an important role in the formation and progression of pancreatic and lung cancers. On the other hand, it is also involved in the development of other tumors such as oral squamous cell carcinoma, bladder and colon (Yan & Theodorescu, 2018). Regarding to the important role of RalA and RalB in tumor growth, the concept of regulation of post translation can lead to be useful in further researches.
Fig. 1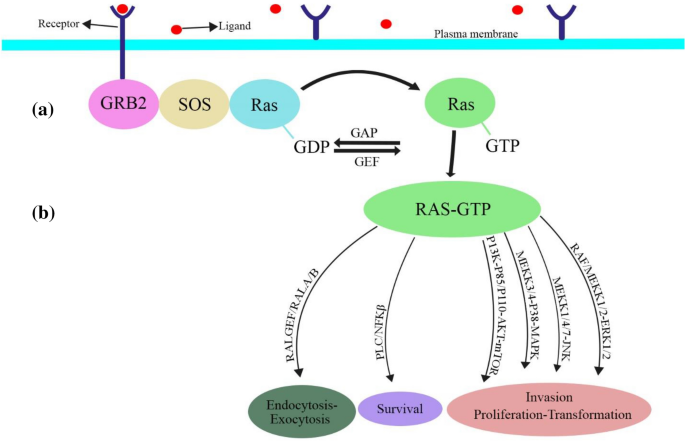
Ras-like activation and downstream effectors. a Ral pathway is activated by RalGEF. b Ras-GTPase regulates critical cellular functions
Full size imageTable 1 Post translational modifications of RalA/RalBFull size tablePTM in proteins extremely interferes with cell signaling pathways. These modifications make cell react to external signals. For example, protein phosphorylation can acts as a binary molecular switch for diffusion of internal signals (Karin & Hunter, 1995). All four Ras proteins that contain G domain are homologous and 20th amino acid sequence at C-terminal called hypervariable region (HVR) (Ehrhardt et al., 2002; Willumsen et al., 1984). Moreover, regulating of Ras activation, via direct GTP binding, has many post translational modifications such as farnesylation, palmitoylation, geranylgeranylation, ubiquitination, phosphorylation, nitrosylation, and acetylation. These modifications can affect Ras exchange, Ras localization, and activation of Ras (Ahearn et al., 2012; Hancock, 2003). To formation of a hydrophobic lipid domain and its binding to the plasma membrane, Ras proteins require farnesylation and palmitoylation at their CAAX terminal (Resh, 2013). These two lipids are essential for repairing Ras protein function (Resh, 2013). Moreover, it has been recorded that many modifications after translation such as lysine acetylation and ubiquitination have effect on the rate of GTP exchange and it leads to interfere with Ras function (Ahearn, Zhou, & Philips, 2018). In the review, the role of Ral signaling in cancer and post translational modifications of Ras proteins have been investigated.
RalGEFs
Ral GTPase is activated by RalGEFs, RalGDS (Ral guanine nucleotide dissociation stimulator), RalGDSlike (RGL, RGL2/Rlf, RGL3), RalGPS1, and RalGPS2 (Kikuchi et al., 1994; Peterson et al., 1996; Shao & Andres, 2000). The activation of Ral by four types of GEFs (RalGDS, RalGDSlike/RGL, RalGDSlike/RGL2, and RGL3) is initiated through Ras dependent signaling (Fig. 1b). Ral-GDS has been activated in a Ras-independent pathway in response to the stimulation of formyl-Met-Leu-Phe (fMLP) receptor that lead to cleaving the inactive β-arrestin-Ral-GDS protein complex. Another two types of GEFs are RalGPS1 and RalGPS2, which do not bind with Ras dependent or Ras-independent proteins (de Bruyn et al., 2000; Martegani et al., 2002; Rebhun et al., 2000). In addition, the activation of RalA by TD-60 (also known as RCC2), a protein that structurally is similar to the Ran GEF RCC1 (but has not GEF activity), makes interaction between microtubules and kinetochore in mitosis (Papini et al., 2015). Disruption of RalGEFs indicates that it has no any overlap in function with different GEFs. Thus, variety in RalGEF activity makes many special characteristics in signaling (inhibition of RalGDS and RalGPS2 leads to disrupt of RalA, while the inhibition of RGL and RalGPS1 disrupts RalB). These disruptions have different effects on cytokinesis in HeLa cells (Cascone et al., 2008). It has been identified that different RalGEFs have non-overlapping and independent functions from Ral in pancreatic cancer cells (Vigil et al., 2010). Recently, one of the most important causes of prostate cancer metastasis, especially to bone, has been attributed to the RalGEFs signaling pathway (Yang et al., 2019). Furthermore, RalGEF and RalGPS2 have been implicated in the mechanism of bone metastasis of pancreatic cancer and survival rates for lung cancer cells, respectively.
RalGAPs
GTP-bound RalA and RalB were inactivated by RalGAPs. RalGAPs consist of heterodimers containing a catalytic α1 or α2 subunit and a β subunit. The molecular identity of RalGAPs have been achieved by molecular characteristics analysis of RalGAPα1 and RalGAPα2 (Emkey et al., 1991; Shirakawa et al., 2009). Heterodimerization of these substrates activates them and regulates RalA and RalB in different ways (Chen et al., 2011). Regarding to the GAP mechanisms, the Serine/Threonine kinase Akt induces RalGAPα1 and RalGAPα2 phosphorylation and thereby Ral activation and also transportation of Glut4 and glucose uptake (Chen et al., 2014; Leto et al., 2013; Oeckinghaus et al., 2014). The RalGAP complex interacts with Ras family GTPase κB-Ras (IκBβ-interacting proteins), which in turn inhibits the Ral function. κB-Ras proteins are one of the major regulators of NF-κB activation pathway. Therefore, it can be noted that these proteins play an important role in the inflammatory responses. These proteins are linked to the Ral-GAP complex and are more essential for their GAP activity against Ral GTPases. It is well established that decrease of the κBRas expression in several human cancers, including pancreatic ductal adenocarcinoma, can provides a growth advantage to tumor cells (Beel et al., 2020; Oeckinghaus et al., 2014). It now appears that, κB-Ras proteins are emerging as a novel tumor suppressor (Oeckinghaus et al., 2014). A study showed that the RalGAPα2, under the influence of some epigenetic mechanisms, led to downregulation of RalGAP and subsequently activated Ral GTPase in oral squamous cell carcinoma and made it more aggressive (Gao et al., 2019). Recent studies have shown that regulation of RalGAP function can affect the Ral activity, and the discovery of regulatory processes may help to know the proliferation and behavior of tumor cells. As result, RalGAPs are potent therapeutic targets in cancers.
Ral Effectors
Ral proteins interact directly with a vast spectrum of downstream effectors. RALBP1 and members of the exocyst complex Sec5 and Exo84 are the most important Ral effectors. RalBP1 is a big protein that has been known as Ral interacting protein also named as RLIP76 and Rip1. RalBP1, with its GTPase activity (Cantor et al., 1995; Jullien-Flores et al., 1995; Park & Weinberg, 1995), helps control the actin dynamics, filopodia formation, and membrane ruffling. It is also a positive regulator for Rac, which is required to induce Ras function (Goldfinger et al., 2006). Regulation of receptor-mediated endocytosis is another function of RalBP1. RalBP1 has interaction with AP2 complex, like POB1 or Reps1, being involved in clathrin endocytosis (Moghadam et al., 2017). Inhibition of these interactions suppresses epidermal growth factor receptors (EGFR) and ionotropic glutamate α-amino-3-hydroxy-5-methyl-4-isoxazolepropionic acid (AMPA) receptor, as well as interferes with various cellular processes such as survival and proliferation (Han et al., 2009; Jullien-Flores et al., 2000; Nakashima et al., 1999). RalBP1 activity is regulated by molecular scaffold (Rossé et al., 2003). During mitosis process, RalBP1 can stimulate mitochondria division by facilitating GTPase-related protein phosphorylation. RalBP1 acts as a ATP-dependent transporter (Awasthi et al., 2000). Research studies have shown that overexpression of RalBP1 has associated with colorectal and breast cancers (Mollberg et al., 2012; Wang et al., 2015). For instance, overexpression of RalBP1 in glioblastoma occurs via activation of Rac1 Jun N-terminal kinase and JNK signaling, which stimulates cell proliferation and survival (Wang et al., 2013). Moreover, RalBP1 induces tumorigenesis phenotypes such as increased glucose uptake, aerobic glycolysis, cell survival, and cell migration. Given the issues raised above, the invasion of cancer cells to other parts of the body is associated with overexpression of this effector (Neel et al., 2012; Wu et al., 2010). Sec5 and Exo84 are other effectors of RalA and RalB. These two proteins are subunits of the octameric exocyst complex that are involved in binding of Golgi secretory vesicles to the plasma membrane before to exocytic fusion (Moskalenko et al., 2002, 2003; Sugihara et al., 2002). The two actions of suppression of RalA and RalB as well intervention in RalA-exocyst function lead to inhibition of the exocyst and breaking of cytokinesis in PC12 cells, respectively (Chen et al., 2007; Kawato et al., 2008; Li et al., 2007; Ljubicic, Bezzi, Vitale, & Regazzi, 2009). In addition, RalA and RalB are dependent on the exocyst and their activities form strong bounds (Hazelett et al., 2011). Previous studies demonstrate that RalA binds to the exocyst complex more effectively than RalB (Fukai et al., 2003; Jin et al., 2005). RalB also stimulates production of autophagosome, which occurs through interacting with Exo84 and thereby activation of ULJ1 (Bodemann et al., 2011). RalB/Sec5 interaction is absolutely necessary to activate TBK1 (Chien et al., 2006). The activation of this kinase has been involved in the innate immune signaling. Ubiquitination of lysine47 inhibits the RalB-Exo84 interaction and stimulates the RalB-Sec5 interplay. Subsequently, the autophagic process of Exo84-Ulk1 has decreased and the survival of Sec5-TBK1-dependent cells and the exocyst complex have increased. This phenotype has been stimulated by mTORC1, which dependent on the interaction between Sec5-Ral and the exocyst complex (Maehama et al., 2008). By activating mTORC, it inhibits autophagy and stimulates cell survival (Camonis & White, 2005; Martin et al., 2014). Therefore, RalB via interaction between Sec5 and Exo84 regulates autophagy and survival. RalB plays a key role in tumor growth using a potential mechanism to regulate autophagy and survival. Interfering with RalA-exocyst interaction leads to a deficiency in prostate tumor cell trafficking (Biondini et al., 2015; Hazelett & Yeaman, 2012). Research studies have shown that inhibition of TBK1 inhibits tumor growth in Ras-induced lung cancers (Zhu et al., 2014). It is important to note that interaction of RalB-TBK1 is essential for the initiation of the tumor (Seguin et al., 2014).
Ral in tumorigenesis
Recently, a number of studies have been focused on Ral function and its importance in tumorigenesis. It is also now clear that Ral plays a role in cancer through the MAPK and PI3K signaling pathways (Urano et al., 1996; White et al., 1996). Research on multiple myeloma has shown that the function and overexpression of Ral is independent of Ras oncogene. Therefore, the study of the Ral mechanism alone could be important in the biology and treatment of cancer (Seibold et al., 2020).
Investigations showed that RalA plays a key role in Ras-induced modifications; so, blocking it may inhibit the growth (Chien & White, 2003; Hahn et al., 1999). Preliminary research has shown that inhibition of RalB induces apoptosis and prevents trafficking in transgenic cells (Oxford et al., 2005). It has been reported for the first time that inhibition of RalB has improved the treatment of Ras mutant metastatic colorectal cancer through the tumor necrosis factor-related apoptosis-inducing ligand (TRAIL) process (Khawaja et al., 2020). RalA is only involved in the initiate of tumor formation, but RalB plays a pivotal role in invasion and metastasis (Lim et al., 2006). According to in vitro studies, inhibition of RalA and RalB prevents tumor initiation and suppression of metastasis in pancreatic cell lines (de Gorter et al., 2008; Song et al., 2015). In another study, melanoma growth was prevented by inhibiting RalA (Győrffy et al., 2015; Martin & Der, 2012; Zipfel et al., 2010). The study conducted on lung cancer found that RalA is more expressed than RalB (Guin et al., 2013; Male et al., 2012). In many studies, overexpression of RalA and RalB have been proven in many cancers (Martin & Der, 2012; Zago et al., 2018). Using of human and mice cells can lead to give provide information about tumorigenesis characteristics. It indicates that there are many physiological and technical differences that can be used in tumorigenesis related studies in human (Martin & Der, 2012).
Inhibition of Ral
Utilization of anti-GGTase-I inhibitors has been widely used for suppress of Ras (Bodemann & White, 2008; Kashatus, 2013; Neel et al., 2011). The inhibition of GGTase-I reduces the ability of glioma cells to trafficking and invasion. So far, the anti tumorigenic effects of these inhibitors, especially in pancreatic cancer, have been well established (Hamada et al., 2011; Lu et al., 2009). The identification of small molecular inhibitors that target directly Ral function can be challengeable. These molecules have several applications in lung cancer (Zinatizadeh et al., 2021; Mohammad Reza Zinatizadeh et al., 2019).
PTM of Ras
Expression of Ras in the endoplasmic reticulum (ER) is temporary, while it is steady in Golgi. Interestingly, activation of Ras is only limited to Golgi and after stimulating of T cell receptor at plasma membrane, it is not recognizable anymore (Bivona et al., 2003; Chiu et al., 2002). This is because activation of Ras in Golgi is done by RasGRP1 (Caloca et al., 2003; Dower et al., 2000; Ebinu et al., 2000).
It has been investigated that PTM in lipid (Farnesylation and palmitoylation) has a directly effect on Ras proteins located at multiple membranes (Apolloni et al., 2000; Choy et al., 1999). Ras is a globular protein that binds to membranes and is regulated by a variety of PTMs. Dynamic modifications of PTM can affect Ras movement and its activities. Ras stability in relation to the membrane facilitates its interaction with GEFs, GAPs, and downstream effects. Not only prenylation, but also other modifications such as phosphorylation, ubiquitination, and acetylation have been reported. As a result, regulation of Ras is highly dependent on PTM (Ahearn et al., 2012).
Prenylation
Prenylation of proteins with CAAX motif leads to binding covalent of farnesyl or geranylgeranyl isoprenoids to conserved cysteine residues at the C-terminal of protein. Farnesyltransferase (FTase) and GGTase-I are enzymes that catalyze them. Lamin B is the first farnesylated protein in mammals that controls cell growth and division (Casey et al., 1989; Farnsworth et al., 1989; Wolda & Glomset, 1988). Geranylgeranyl can also bind to proteins (Farnsworth et al., 1990). Overall prenylation of Ras is required to modifying oncogene in fibroblast cells (Kohl et al., 1993).
Obstruction of Frenzil transmission by the Faranzyl transferase (FTI) inhibitor prevents Ras signaling in cancers (Siegel-Lakhai et al., 2005; Sparano et al., 2006, 2009). FTase contains a binding site for CAAX box (Lerner et al., 1995; Sun et al., 1995). Prenylation replacement called geranylgeranylation that is found in N-Ras and K-Ras but not in H-Ras (Crespo, Ohkanda, Yen, Hamilton, & Sebti, 2001; Whyte et al., 1997). It has been revealed that GGTase-I deficiency reduces tumor formation and improves survival in mice with K-Ras-induced lung cancer (Sjogren et al., 2007). Studies demonstrated that FTase and GGTase-I targeting in mice decrease Lung carcinogenesis, thereby the lifespan in these mice increases significantly. Thus inhibition of FTase and GGTase-I can prevent occurring cancer. Overall inhibitors, which can target these two enzymes, can be an effective strategy for preventing of K-Ras signaling pathway (Liu et al., 2010; Lobell et al., 2002).
RCE1 is very important in Ras movement (Kim et al., 1999). Suppression of RCE1 in fibroblasts leads to inhibition of cell growth and reduction of Ras-induced modifications (Bergo et al., 2002).
Several studies have shown that inhibition of ICMT reduced growth in K-Ras-induced cancers (Bergo et al., 2004; Wahlstrom et al., 2008). The anticancer drug methotrexate increases homocysteine levels, thereby increasing hypomethylation in cells and significantly reducing Ras methylation (Winter-Vann et al., 2003). Some ICMT inhibitors have been designed to induce apoptosis and decreases tumor growth (Wang, Hossain, et al., 2010; Wang, Owens, et al., 2010).
Phosphorylation
The activity of K-Ras4B proteins can be decreased by phosphorylation of S181. This phosphorylation stimulates segregation of K-Ras4B from plasma membrane to ER (Ballester et al., 1987; Bivona et al., 2006). The importance of this phosphorylation had not been revealed until 2006. However, in 2006, scientists demonstrated that phosphorylation of S181 in K-Ras4 accrues via activation of PKC and Phorbol 12-myristate 13-acetate (PMA) (Bivona et al., 2006). They proved that after temporary agitation of PKC-α, K-Ras4B is immediately phosphorylated at the S181 position, leading to its transfer from the plasma membrane to the inner membrane of the ER, Golgi, and mitochondria. Moreover, transferring of phosphorylated K-Ras4B to the mitochondria outer membrane stimulates apoptosis. Previous studies have shown that decreased PKC activity in fibroblasts leads to cell apoptosis (Xia et al., 2007). Expression of v-H-Ras and v-K-Ras in different cells caused apoptosis and also inhibited PKC activity (Chen & Faller, 1995). Thus stimulating or inhibiting of PKC maybe leads to make K-Ras-induced apoptosis. Calmodulin, presents in eukaryotic cells inhibits Ras, thereby reducing the activity of the Ras-Raf-MEK-ERK pathways. Binding of calmodulin to Ras has been shown to inhibit K-Ras4B activity (Alvarez-Moya et al., 2010). C-terminal of HVR in K-Ras consists of S181 residues and farnesyl group that are required for binding calmodulin. Moreover, phosphorylation of S181 disrupts K-Ras associated plasma membrane (Sidhu et al., 2003).
Ubiquitination
The main activities of F-Box protein include stimulation of polyubiquitination, disruption of Ras isoforms, and inhibition of transformation (Kim et al., 2009). Also Wnt signaling causes induced polyubiquitination and increase Ras activation (Jeong et al., 2012; Shukla et al., 2014). H-Ras and N-Ras have been reported to be diubiquitinated in a lysine residue, while K-Ras resists these modifications (Jura et al., 2006). Ubiquitination of Ras inhibits endosomal Ras and prevents activation of ERK (Xu et al., 2010; Yan et al., 2010). Ras can also be activated via ubiquitination (Sasaki et al., 2011). Monoubiquitination of K-Ras at the K147 position increases its oncogenicity. Ubiquitination of K147 in Ras extremely inhibits hydrolysis by GAP and leads to activate Ras (Baker et al., 2013). H-Ras mostly is activated by ubiquitination at K117 and it leads to accelerate nucleotide exchange and increases GTP loading. Interestingly, there are just a few ubiquitinations at K147 that can cause important biological consequences. These results indicate that different Ras isoforms in many regions is monoubiquitinated. After ubiquitination of Ras, many mechanisms occur to modulate Ras in different tissues and cells and also its isoforms (Baker et al., 2013).
Acetylation
In addition of ubiquitination, wild-mutant type of Ras is acetylated at K104 (Yang et al., 2012), and it leads to reducing transformation capacity of K-Ras. In cancer cells, HDAC6 and SIRT2 induce deacetylating in Ras; in contrast, HDAC6 and SirT2 inactivity cause reduction in cell survival of NIH3T3 with K-RasG12V expression, but has not any effect on cells with K-Ras G12V/K104A expression (Yang et al., 2013). These results show that HDAC6 and SIRT2 targeting maybe effective in cancer patients.
S-nitrosylation
S-nitrosylation is a redox PTM that is formed by a covalent bond between nitric oxide (NO) and reactive cysteine residues (Aranda, Lopez-Pedrera, De La Haba-Rodriguez, & Rodriguez-Ariza, 2012; Halloran, Parakh, & Atkin, 2013; Monteiro, Costa, Reis, & Stern, 2015; Tang, Wei, & Liu, 2012). NO can be synthesis via different nitric oxide synthase (NOS) isoforms in body.
The mechanism that has been described for this modification is when Ras proteins associate with NO, is nitrosylated at C118 (Lander et al., 1997; Williams et al., 2003). This region is extremely conserved in all of the Ras isoforms. C118 can have a direct interplay with nitrogen dioxide radical or glutathionyl radical, this change causes increasing of (G) nucleotide exchange, stimulating Ras activity and activation of MAPK pathways (Heo & Campbell, 2004; Lander et al., 1996). In Ras, S-nitrosylation without any mutation stimulates tumor growth in pancreatic. Activation of NOS leads to increase NO production and stimulates pancreatic tumor cell growth via activating of wild-type Ras (Lim et al., 2008). NO activates Ras/MEK/ERK signaling pathway at estrogen-receptor in breast cancer and thereby induces phosphorylation and activation of Est-1 translation (Switzer et al., 2012). Translation factor of Est-1 also regulates translation of other genes, which interfere in tumor development and metastasis.
It has also been shown that nitrosylation of Ras is denitrosylated by GSNO. Denitrosylation of Ras leads to its increased activity. In human lung cancer, the activity and the expression level of GSNO decreased. Thus decreased activity of GSNO has an effect on developing lung cancer in cigarette smoker (Marozkina et al., 2012).
Conclusion
There had been several studies on the effect of RalA and RalB function on embryo tumor growth at downstream of Ras that cause the inhibition of these signaling pathways provide a strong therapeutic approach to cancer treatment. However, over the past 30 years, trials for inhibition of Ral by Ras inhibitors have been associated with many problems. There is still a long way to go to fully understand how GTPases involved in Ras-induced tumor growth. The development of new tools to study of these proteins, including new drug inhibitors and genetic advances, will finally allow us to pose key questions such as how to use the effector and role of different RalGEFs, and how to study key pathways of different RalA and RalB tumorigenesis activities using mouse models. If these questions are answered, we will come up with a set of new strategies to treat Ras-induced malignant tumors.
Ras proteins has a binary function to transmission different extracellular messages into intracellular signaling network. It has been proved that Ras proteins associate with different plasma membranes. Although all Ras isoforms have been found at plasma membrane, H-Ras and N-Ras are located in Golgi, and K-Ras at ER and mitochondria outer membrane. Ras proteins in Golgi lead to activate MAPK pathway, which is essential for development of T cells population. It seems that activating of Ras in plasma membrane is fast and temporary but this happens with delay and more stable in Golgi. Ras functions are related with PTM of Ras proteins. Previous studies indicated that Ras activates the MAPK pathway. Indeed some demonstrated that HVR region is required for dimerization and activating of Ras. Also, prenylation has a pivotal role for Ras dimerization.
Direct inhibiting of Ras by drug is because of lack of proper binding site in Ras proteins. An alternative approach is Ras PTM that prevents Ras binding to cellular membrane. Development of binary inhibitors that target FTase and GGTase-I are extremely required to deactivate Ras. Targeting of interaction between Ras and PDEδ prenylation binding protein has been offered as an alternative approach for deactivated Ras. Overall a comprehensive information of Ras modifications can help to design useful inhibitors for disrupting of Ras signaling.
References
Ahearn, I. M., Haigis, K., Bar-Sagi, D., & Philips, M. R. (2012). Regulating the regulator: Post-translational modification of RAS. Nature Reviews Molecular Cell Biology, 13(1), 39–51.
Ahearn, I., Zhou, M., & Philips, M. R. (2018). Posttranslational modifications of RAS proteins. Cold Spring Harbor Perspectives in Medicine, 8(11), a031484.
Alvarez-Moya, B., Lopez-Alcala, C., Drosten, M., Bachs, O., & Agell, N. (2010). K-Ras4B phosphorylation at Ser181 is inhibited by calmodulin and modulates K-Ras activity and function. Oncogene, 29(44), 5911–5922.
Apolloni, A., Prior, I. A., Lindsay, M., Parton, R. G., & Hancock, J. F. (2000). H-ras but not K-ras traffics to the plasma membrane through the exocytic pathway. Molecular and Cellular Biology, 20(7), 2475–2487.
Aranda, E., Lopez-Pedrera, C., De La Haba-Rodriguez, R. J., & Rodriguez-Ariza, A. (2012). Nitric oxide and cancer: The emerging role of S-nitrosylation. Current Molecular Medicine, 12(1), 50–67.
Awasthi, S., Cheng, J., Singhal, S. S., Saini, M. K., Pandya, U., Pikula, S., Awasthi, Y. C., et al. (2000). Novel function of human RLIP76: ATP-dependent transport of glutathione conjugates and doxorubicin. Biochemistry, 39(31), 9327–9334.
Baker, R., Wilkerson, E. M., Sumita, K., Isom, D. G., Sasaki, A. T., Dohlman, H. G., & Campbell, S. L. (2013). Differences in the regulation of K-Ras and H-Ras isoforms by monoubiquitination. Journal of Biological Chemistry, 288(52), 36856–36862.
Ballester, R., Furth, M., & Rosen, O. (1987). Phorbol ester-and protein kinase C-mediated phosphorylation of the cellular Kirsten ras gene product. Journal of Biological Chemistry, 262(6), 2688–2695.
Beel, S., Kolloch, L., Apken, L. H., Jürgens, L., Bolle, A., Sudhof, N., Steinestel, K., et al. (2020). κB-Ras and Ral GTPases regulate acinar to ductal metaplasia during pancreatic adenocarcinoma development and pancreatitis. Nature Communications, 11(1), 1–16.
Bergo, M. O., Ambroziak, P., Gregory, C., George, A., Otto, J. C., Kim, E., Young, S. G., et al. (2002). Absence of the CAAX endoprotease Rce1: Effects on cell growth and transformation. Molecular and Cellular Biology, 22(1), 171–181.
Bergo, M. O., Gavino, B. J., Hong, C., Beigneux, A. P., McMahon, M., Casey, P. J., & Young, S. G. (2004). Inactivation of Icmt inhibits transformation by oncogenic K-Ras and B-Raf. The Journal of Clinical Investigation, 113(4), 539–550.
Biondini, M., Duclos, G., Meyer-Schaller, N., Silberzan, P., Camonis, J., & Parrini, M. C. (2015). RalB regulates contractility-driven cancer dissemination upon TGFβ stimulation via the RhoGEF GEF-H1. Scientific Reports, 5(1), 1–14.
Bivona, T. G., De Castro, I. P., Ahearn, I. M., Grana, T. M., Chiu, V. K., Lockyer, P. J., Philips, M. R., et al. (2003). Phospholipase Cγ activates Ras on the Golgi apparatus by means of RasGRP1. Nature, 424(6949), 694–698.
Bivona, T. G., Quatela, S. E., Bodemann, B. O., Ahearn, I. M., Soskis, M. J., Mor, A., Saba, S. G., et al. (2006). PKC regulates a farnesyl-electrostatic switch on K-Ras that promotes its association with Bcl-XL on mitochondria and induces apoptosis. Molecular Cell, 21(4), 481–493.
Bodemann, B. O., Orvedahl, A., Cheng, T., Ram, R. R., Ou, Y.-H., Formstecher, E., Balakireva, M., et al. (2011). RalB and the exocyst mediate the cellular starvation response by direct activation of autophagosome assembly. Cell, 144(2), 253–267.
Bodemann, B. O., & White, M. A. (2008). Ral GTPases and cancer: Linchpin support of the tumorigenic platform. Nature Reviews Cancer, 8(2), 133–140.
Caloca, M. J., Zugaza, J. L., & Bustelo, X. R. (2003). Exchange factors of the RasGRP family mediate Ras activation in the Golgi. Journal of Biological Chemistry, 278(35), 33465–33473.
Camonis, J. H., & White, M. A. (2005). Ral GTPases: Corrupting the exocyst in cancer cells. Trends in Cell Biology, 15(6), 327–332.
Cantor, S. B., Urano, T., & Feig, L. A. (1995). Identification and characterization of Ral-binding protein 1, a potential downstream target of Ral GTPases. Molecular and Cellular Biology, 15(8), 4578–4584.
Cascone, I., Selimoglu, R., Ozdemir, C., Del Nery, E., Yeaman, C., White, M., & Camonis, J. (2008). Distinct roles of RalA and RalB in the progression of cytokinesis are supported by distinct RalGEFs. The EMBO Journal, 27(18), 2375–2387.
Casey, P. J., Solski, P. A., Der, C. J., & Buss, J. E. (1989). p21ras is modified by a farnesyl isoprenoid. Proceedings of the National Academy of Sciences, 86(21), 8323–8327.
Chen, C. Y., & Faller, D. V. (1995). Direction of p21ras-generated signals towards cell growth or apoptosis is determined by protein kinase C and Bcl-2. Oncogene, 11(8), 1487–1498.
Chen, Q., Quan, C., Xie, B., Chen, L., Zhou, S., Toth, R., Horiuchi, H., et al. (2014). GARNL1, a major RalGAP α subunit in skeletal muscle, regulates insulin-stimulated RalA activation and GLUT4 trafficking via interaction with 14-3-3 proteins. Cellular Signalling, 26(8), 1636–1648.
Chen, X.-W., Inoue, M., Hsu, S. C., & Saltiel, A. R. (2006). RalA-exocyst-dependent recycling endosome trafficking is required for the completion of cytokinesis. Journal of Biological Chemistry, 281(50), 38609–38616.
Chen, X.-W., Leto, D., Chiang, S.-H., Wang, Q., & Saltiel, A. R. (2007). Activation of RalA is required for insulin-stimulated Glut4 trafficking to the plasma membrane via the exocyst and the motor protein Myo1c. Developmental Cell, 13(3), 391–404.
Chen, X.-W., Leto, D., Xiong, T., Yu, G., Cheng, A., Decker, S., & Saltiel, A. R. (2011). A Ral GAP complex links PI 3-kinase/Akt signaling to RalA activation in insulin action. Molecular Biology of the Cell, 22(1), 141–152.
Chien, Y., Kim, S., Bumeister, R., Loo, Y.-M., Kwon, S. W., Johnson, C. L., Gale, M., Jr., et al. (2006). RalB GTPase-mediated activation of the IκB family kinase TBK1 couples innate immune signaling to tumor cell survival. Cell, 127(1), 157–170.
Chien, Y., & White, M. A. (2003). RAL GTPases are linchpin modulators of human tumour-cell proliferation and survival. EMBO Reports, 4(8), 800–806.
Chiu, V. K., Bivona, T., Hach, A., Sajous, J. B., Silletti, J., Wiener, H., Philips, M. R., et al. (2002). Ras signalling on the endoplasmic reticulum and the Golgi. Nature Cell Biology, 4(5), 343–350.
Choy, E., Chiu, V. K., Silletti, J., Feoktistov, M., Morimoto, T., Michaelson, D., Philips, M. R., et al. (1999). Endomembrane trafficking of ras: The CAAX motif targets proteins to the ER and Golgi. Cell, 98(1), 69–80.
Crespo, N. C., Ohkanda, J., Yen, T. J., Hamilton, A. D., & Sebti, S. D. M. (2001). The farnesyltransferase inhibitor, FTI-2153, blocks bipolar spindle formation and chromosome alignment and causes prometaphase accumulation during mitosis of human lung cancer cells. Journal of Biological Chemistry, 276(19), 16161–16167.
de Bruyn, K. M., de Rooij, J., Wolthuis, R. M., Rehmann, H., Wesenbeek, J., Cool, R. H., Bos, J. L., et al. (2000). RalGEF2, a pleckstrin homology domain containing guanine nucleotide exchange factor for Ral. Journal of Biological Chemistry, 275(38), 29761–29766.
de Gorter, D. J., Reijmers, R. M., Beuling, E. A., Naber, H. P., Kuil, A., Kersten, M. J., Spaargaren, M., et al. (2008). The small GTPase Ral mediates SDF-1–induced migration of B cells and multiple myeloma cells. Blood, the Journal of the American Society of Hematology, 111(7), 3364–3372.
Dower, N. A., Stang, S. L., Bottorff, D. A., Ebinu, J. O., Dickie, P., Ostergaard, H. L., & Stone, J. C. (2000). RasGRP is essential for mouse thymocyte differentiation and TCR signaling. Nature Immunology, 1(4), 317–321.
Ebinu, J. O., Stang, S. L., Teixeira, C., Bottorff, D. A., Hooton, J., Blumberg, P. M., Stone, J. C., et al. (2000). RasGRP links T-cell receptor signaling to Ras. Blood, the Journal of the American Society of Hematology, 95(10), 3199–3203.
Ehrhardt, A., Ehrhardt, G. R., Guo, X., & Schrader, J. W. (2002). Ras and relatives—job sharing and networking keep an old family together. Experimental Hematology, 30(10), 1089–1106.
Emkey, R., Freedman, S., & Feig, L. (1991). Characterization of a GTPase-activating protein for the Ras-related Ral protein. Journal of Biological Chemistry, 266(15), 9703–9706.
Falsetti, S. C., Wang, D.-A., Peng, H., Carrico, D., Cox, A. D., Der, C. J., Sebti, S. M., et al. (2007). Geranylgeranyltransferase I inhibitors target RalB to inhibit anchorage-dependent growth and induce apoptosis and RalA to inhibit anchorage-independent growth. Molecular and Cellular Biology, 27(22), 8003–8014.
Farnsworth, C. C., Gelb, M. H., & Glomset, J. A. (1990). Identification of geranylgeranyl-modified proteins in HeLa cells. Science, 247(4940), 320–322.
Farnsworth, C. C., Wolda, S. L., Gelb, M. H., & Glomset, J. A. (1989). Human lamin B contains a farnesylated cysteine residue. Journal of Biological Chemistry, 264(34), 20422–20429.
Fernández, R. M., Ruiz-Miró, M., Dolcet, X., Aldea, M., & Garí, E. (2011). Cyclin D1 interacts and collaborates with Ral GTPases enhancing cell detachment and motility. Oncogene, 30(16), 1936–1946.
Fruman, D. A., & Rommel, C. (2014). PI3K and cancer: Lessons, challenges and opportunities. Nature Reviews Drug Discovery, 13(2), 140–156.
Fukai, S., Matern, H. T., Jagath, J. R., Scheller, R. H., & Brunger, A. T. (2003). Structural basis of the interaction between RalA and Sec5, a subunit of the sec6/8 complex. The EMBO Journal, 22(13), 3267–3278.
Gao, P., Liu, S., Yoshida, R., Shi, C., Yoshimachi, S., Sakata, N., Nakayama, H., et al. (2019). Ral GTPase activation by downregulation of RalGAP enhances oral squamous cell carcinoma progression. Journal of Dental Research, 98(9), 1011–1019.
Gentry, L. R., Martin, T. D., Reiner, D. J., & Der, C. J. (2014). Ral small GTPase signaling and oncogenesis: more than just 15 minutes of fame. Biochimica Et Biophysica Acta (BBA)-Molecular Cell Research, 1843(12), 2976–2988.
Gentry, L. R., Nishimura, A., Cox, A. D., Martin, T. D., Tsygankov, D., Nishida, M., Der, C. J., et al. (2015). Divergent roles of CAAX motif-signaled posttranslational modifications in the regulation and subcellular localization of Ral GTPases. Journal of Biological Chemistry, 290(37), 22851–22861.
Goldfinger, L. E., Ptak, C., Jeffery, E. D., Shabanowitz, J., Hunt, D. F., & Ginsberg, M. H. (2006). RLIP76 (RalBP1) is an R-Ras effector that mediates adhesion-dependent Rac activation and cell migration. The Journal of Cell Biology, 174(6), 877–888.
Guin, S., Ru, Y., Wynes, M. W., Mishra, R., Lu, X., Owens, C., Kern, J. A., et al. (2013). Contributions of KRAS and RAL in non–small-cell lung cancer growth and progression. Journal of Thoracic Oncology, 8(12), 1492–1501.
Győrffy, B., Stelniec-Klotz, I., Sigler, C., Kasack, K., Redmer, T., Qian, Y., & Schäfer, R. (2015). Effects of RAL signal transduction in KRAS-and BRAF-mutated cells and prognostic potential of the RAL signature in colorectal cancer. Oncotarget, 6(15), 13334.
Hahn, W. C., Counter, C. M., Lundberg, A. S., Beijersbergen, R. L., Brooks, M. W., & Weinberg, R. A. (1999). Creation of human tumour cells with defined genetic elements. Nature, 400(6743), 464–468.
Halloran, M., Parakh, S., & Atkin, J. (2013). The role of s-nitrosylation and s-glutathionylation of protein disulphide isomerase in protein misfolding and neurodegeneration. International Journal of Cell Biology, 2013, 5.
Hamada, M., Miki, T., Iwai, S., Shimizu, H., & Yura, Y. (2011). Involvement of RhoA and RalB in geranylgeranyltransferase I inhibitor-mediated inhibition of proliferation and migration of human oral squamous cell carcinoma cells. Cancer Chemotherapy and Pharmacology, 68(3), 559–569.
Han, K., Kim, M.-H., Seeburg, D., Seo, J., Verpelli, C., Han, S., Kim, K., et al. (2009). Regulated RalBP1 binding to RalA and PSD-95 controls AMPA receptor endocytosis and LTD. PLoS Biology, 7(9), e1000187.
Hancock, J. F. (2003). Ras proteins: Different signals from different locations. Nature Reviews Molecular Cell Biology, 4(5), 373–385.
Hazelett, C. C., Sheff, D., & Yeaman, C. (2011). RalA and RalB differentially regulate development of epithelial tight junctions. Molecular Biology of the Cell, 22(24), 4787–4800.
Hazelett, C. C., & Yeaman, C. (2012). Sec5 and Exo84 mediate distinct aspects of RalA-dependent cell polarization. PLoS ONE, 7(6), e39602.
Heo, J., & Campbell, S. L. (2004). Mechanism of p21Ras S-nitrosylation and kinetics of nitric oxide-mediated guanine nucleotide exchange. Biochemistry, 43(8), 2314–2322.
Jeong, W.-J., Yoon, J., Park, J.-C., Lee, S.-H., Lee, S.-H., Kaduwal, S., Choi, K.-Y., et al. (2012). Ras stabilization through aberrant activation of Wnt/β-catenin signaling promotes intestinal tumorigenesis. Science Signaling, 5(219), ra30–ra31.
Jin, R., Junutula, J. R., Matern, H. T., Ervin, K. E., Scheller, R. H., & Brunger, A. T. (2005). Exo84 and Sec5 are competitive regulatory Sec6/8 effectors to the RalA GTPase. The EMBO Journal, 24(12), 2064–2074.
Jullien-Flores, V., Dorseuil, O., Romero, F., Letourneur, F., Saragosti, S., Berger, R., Camonis, J. H., et al. (1995). Bridging Ral GTPase to Rho pathways: RLIP76, a Ral effector with CDC42/Rac GTPase-activating protein activity. Journal of Biological Chemistry, 270(38), 22473–22477.
Jullien-Flores, V., Mahé, Y., Mirey, G., Leprince, C., Meunier-Bisceuil, B., Sorkin, A., & Camonis, J. H. (2000). RLIP76, an effector of the GTPase Ral, interacts with the AP2 complex: Involvement of the Ral pathway in receptor endocytosis. Journal of Cell Science, 113(16), 2837–2844.
Jura, N., Scotto-Lavino, E., Sobczyk, A., & Bar-Sagi, D. (2006). Differential modification of Ras proteins by ubiquitination. Molecular Cell, 21(5), 679–687.
Karin, M., & Hunter, T. (1995). Transcriptional control by protein phosphorylation: Signal transmission from the cell surface to the nucleus. Current Biology, 5(7), 747–757.
Kashatus, D. F. (2013). Ral GTPases in tumorigenesis: Emerging from the shadows. Experimental Cell Research, 319(15), 2337–2342.
Kawato, M., Shirakawa, R., Kondo, H., Higashi, T., Ikeda, T., Okawa, K., Horiuchi, H., et al. (2008). Regulation of platelet dense granule secretion by the Ral GTPase-exocyst pathway. Journal of Biological Chemistry, 283(1), 166–174.
Khawaja, H., Campbell, A., Roberts, J. Z., Javadi, A., O’Reilly, P., McArt, D., Bardelli, A., et al. (2020). RALB GTPase: A critical regulator of DR5 expression and TRAIL sensitivity in KRAS mutant colorectal cancer. Cell Death & Disease, 11(10), 1–18.
Kikuchi, A., Demo, S. D., Ye, Z.-H., Chen, Y.-W., & Williams, L. T. (1994). ralGDS family members interact with the effector loop of ras p21. Molecular and Cellular Biology, 14(11), 7483–7491.
Kim, E., Ambroziak, P., Otto, J. C., Taylor, B., Ashby, M., Shannon, K., Young, S. G., et al. (1999). Disruption of the mouse Rce1 gene results in defective Ras processing and mislocalization of Ras within cells. Journal of Biological Chemistry, 274(13), 8383–8390.
Kim, S.-E., Yoon, J.-Y., Jeong, W.-J., Jeon, S.-H., Park, Y., Yoon, J.-B., Choi, K.-Y., et al. (2009). H-Ras is degraded by Wnt/β-catenin signaling via β-TrCP-mediated polyubiquitylation. Journal of Cell Science, 122(6), 842–848.
Kohl, N. E., Mosser, S. D., DeSolms, S. J., Giuliani, E. A., Pompliano, D. L., Graham, S. L., Gibbs, J. B., et al. (1993). Selective inhibition of ras-dependent transformation by a farnesyltransferase inhibitor. Science, 260(5116), 1934–1937.
Lander, H. M., Hajjar, D. P., Hempstead, B. L., Mirza, U. A., Chait, B. T., Campbell, S., & Quilliam, L. A. (1997). A molecular redox switch on p21ras: Structural basis for the nitric oxide-p21ras interaction. Journal of Biological Chemistry, 272(7), 4323–4326.
Lander, H. M., Milbank, A. J., Tauras, J. M., Hajjar, D. P., Hempstead, B. L., Schwartz, G. D., Burk, S. C., et al. (1996). Redox regulation of cell signalling. Nature, 381(6581), 380–381.
Lerner, E. C., Qian, Y., Blaskovich, M. A., Fossum, R. D., Vogt, A., Sun, J., Sebti, S. M., et al. (1995). Ras CAAX peptidomimetic FTI-277 selectively blocks oncogenic Ras signaling by inducing cytoplasmic accumulation of inactive Ras-Raf complexes. Journal of Biological Chemistry, 270(45), 26802–26806.
Leto, D., Uhm, M., Williams, A., Chen, X.-W., & Saltiel, A. R. (2013). Negative regulation of the RalGAP complex by 14-3-3. Journal of Biological Chemistry, 288(13), 9272–9283.
Li, G., Han, L., Chou, T.-C., Fujita, Y., Arunachalam, L., Xu, A., Wang, L., et al. (2007). RalA and RalB function as the critical GTP sensors for GTP-dependent exocytosis. Journal of Neuroscience, 27(1), 190–202.
Lim, K.-H., Ancrile, B. B., Kashatus, D. F., & Counter, C. M. (2008). Tumour maintenance is mediated by eNOS. Nature, 452(7187), 646–649.
Lim, K.-H., Brady, D. C., Kashatus, D. F., Ancrile, B. B., Der, C. J., Cox, A. D., & Counter, C. M. (2010). Aurora-A phosphorylates, activates, and relocalizes the small GTPase RalA. Molecular and Cellular Biology, 30(2), 508–523.
Lim, K.-H., O’Hayer, K., Adam, S. J., Kendall, S. D., Campbell, P. M., Der, C. J., & Counter, C. M. (2006). Divergent roles for RalA and RalB in malignant growth of human pancreatic carcinoma cells. Current Biology, 16(24), 2385–2394.
Liu, M., Sjogren, A.-K.M., Karlsson, C., Ibrahim, M. X., Andersson, K. M., Olofsson, F. J., Chen, Z., et al. (2010). Targeting the protein prenyltransferases efficiently reduces tumor development in mice with K-RAS-induced lung cancer. Proceedings of the National Academy of Sciences, 107(14), 6471–6476.
Ljubicic, S., Bezzi, P., Vitale, N., & Regazzi, R. (2009). The GTPase RalA regulates different steps of the secretory process in pancreatic β-cells. PLoS ONE, 4(11), e7770.
Lobell, R. B., Liu, D., Buser, C. A., Davide, J. P., DePuy, E., Hamilton, K., Motzel, S. L., et al. (2002). Preclinical and clinical pharmacodynamic assessment of L-778,123, a dual inhibitor of farnesyl: Protein transferase and geranylgeranyl: Protein transferase type-I. Molecular Cancer Therapeutics, 1(9), 747–758.
Lu, J., Chan, L., Fiji, H. D., Dahl, R., Kwon, O., & Tamanoi, F. (2009). In vivo antitumor effect of a novel inhibitor of protein geranylgeranyltransferase-I. Molecular Cancer Therapeutics, 8(5), 1218–1226.
Maehama, T., Tanaka, M., Nishina, H., Murakami, M., Kanaho, Y., & Hanada, K. (2008). RalA functions as an indispensable signal mediator for the nutrient-sensing system. Journal of Biological Chemistry, 283(50), 35053–35059.
Male, H., Patel, V., Jacob, M. A., Borrego-Diaz, E., Wang, K., Young, D. A., O’Brien-Ladner, A., et al. (2012). Inhibition of RalA signaling pathway in treatment of non-small cell lung cancer. Lung Cancer, 77(2), 252–259.
Marozkina, N. V., Wei, C., Yemen, S., Wallrabe, H., Nagji, A. S., Liu, L., Gaston, B., et al. (2012). S-nitrosoglutathione reductase in human lung cancer. American Journal of Respiratory Cell and Molecular Biology, 46(1), 63–70.
Martegani, E., Ceriani, M., Tisi, R., & Berruti, G. (2002). Cloning and characterization of a new Ral-GEF expressed in mouse testis. Annals of the New York Academy of Sciences, 973(1), 135–137.
Martin, T. D., Chen, X.-W., Kaplan, R. E., Saltiel, A. R., Walker, C. L., Reiner, D. J., & Der, C. J. (2014). Ral and Rheb GTPase activating proteins integrate mTOR and GTPase signaling in aging, autophagy, and tumor cell invasion. Molecular Cell, 53(2), 209–220.
Martin, T. D., & Der, C. J. (2012). Differential involvement of RalA and RalB in colorectal cancer. Small GTPases, 3(2), 126–130.
Martin, T. D., Mitin, N., Cox, A. D., Yeh, J. J., & Der, C. J. (2012). Phosphorylation by protein kinase Cα regulates RalB small GTPase protein activation, subcellular localization, and effector utilization. Journal of Biological Chemistry, 287(18), 14827–14836.
McLaughlin, S., & Aderem, A. (1995). The myristoyl-electrostatic switch: A modulator of reversible protein-membrane interactions. Trends in Biochemical Sciences, 20(7), 272–276.
Michaelson, D., Ali, W., Chiu, V. K., Bergo, M., Silletti, J., Wright, L., Philips, M., et al. (2005). Postprenylation CAAX processing is required for proper localization of Ras but not Rho GTPases. Molecular Biology of the Cell, 16(4), 1606–1616.
Moghadam, A. R., Patrad, E., Tafsiri, E., Peng, W., Fangman, B., Pluard, T. J., Ricke, B., et al. (2017). Ral signaling pathway in health and cancer. Cancer Medicine, 6(12), 2998–3013.
Mollberg, N., Steinert, G., Aigner, M., Hamm, A., Lin, F.-J., Elbers, H., Koch, M., et al. (2012). Overexpression of RalBP1 in colorectal cancer is an independent predictor of poor survival and early tumor relapse. Cancer Biology & Therapy, 13(8), 694–700.
Monteiro, H., Costa, P., Reis, A., & Stern, A. (2015). Nitric oxide: protein tyrosine phosphorylation and protein S-nitrosylation in cancer. Biomedical Journal, 38, 5.
Moskalenko, S., Henry, D. O., Rosse, C., Mirey, G., Camonis, J. H., & White, M. A. (2002). The exocyst is a Ral effector complex. Nature Cell Biology, 4(1), 66–72.
Moskalenko, S., Tong, C., Rosse, C., Mirey, G., Formstecher, E., Daviet, L., White, M. A., et al. (2003). Ral GTPases regulate exocyst assembly through dual subunit interactions. Journal of Biological Chemistry, 278(51), 51743–51748.
Nakashima, S., Morinaka, K., Koyama, S., Ikeda, M., Kishida, M., Okawa, K., Kikuchi, A., et al. (1999). Small G protein Ral and its downstream molecules regulate endocytosis of EGF and insulin receptors. The EMBO Journal, 18(13), 3629–3642.
Neel, N. F., Martin, T. D., Stratford, J. K., Zand, T. P., Reiner, D. J., & Der, C. J. (2011). The RalGEF-Ral effector signaling network: The road less traveled for anti-Ras drug discovery. Genes & Cancer, 2(3), 275–287.
Neel, N. F., Rossman, K. L., Martin, T. D., Hayes, T. K., Yeh, J. J., & Der, C. J. (2012). The RalB small GTPase mediates formation of invadopodia through a GTPase-activating protein-independent function of the RalBP1/RLIP76 effector. Molecular and Cellular Biology, 32(8), 1374–1386.
Neyraud, V., Aushev, V. N., Hatzoglou, A., Meunier, B., Cascone, I., & Camonis, J. (2012). RalA and RalB proteins are ubiquitinated GTPases, and ubiquitinated RalA increases lipid raft exposure at the plasma membrane. Journal of Biological Chemistry, 287(35), 29397–29405.
Nishimura, A., & Linder, M. E. (2013). Identification of a novel prenyl and palmitoyl modification at the CaaX motif of Cdc42 that regulates RhoGDI binding. Molecular and Cellular Biology, 33(7), 1417–1429.
Oeckinghaus, A., Postler, T. S., Rao, P., Schmitt, H., Schmitt, V., Grinberg-Bleyer, Y., Ghosh, S., et al. (2014). κB-Ras proteins regulate both NF-κB-dependent inflammation and Ral-dependent proliferation. Cell Reports, 8(6), 1793–1807.
Oxford, G., Owens, C. R., Titus, B. J., Foreman, T. L., Herlevsen, M. C., Smith, S. C., & Theodorescu, D. (2005). RalA and RalB: Antagonistic relatives in cancer cell migration. Cancer Research, 65(16), 7111–7120.
Papini, D., Langemeyer, L., Abad, M. A., Kerr, A., Samejima, I., Eyers, P. A., Earnshaw, W. C., et al. (2015). TD-60 links RalA GTPase function to the CPC in mitosis. Nature Communications, 6(1), 1–12.
Park, S.-H., & Weinberg, R. A. (1995). A putative effector of Ral has homology to Rho/Rac GTPase activating proteins. Oncogene, 11(11), 2349–2355.
Peterson, S. N., Trabalzini, L., Brtva, T. R., Fischer, T., Altschuler, D. L., Martelli, P., White, G. C., II., et al. (1996). Identification of a novel RalGDS-related protein as a candidate effector for Ras and Rap1. Journal of Biological Chemistry, 271(47), 29903–29908.
Rebhun, J. F., Chen, H., & Quilliam, L. A. (2000). Identification and characterization of a new family of guanine nucleotide exchange factors for the ras-related GTPase Ral. Journal of Biological Chemistry, 275(18), 13406–13410.
Resh, M. D. (2013). Covalent lipid modifications of proteins. Current Biology, 23(10), R431–R435.
Rossé, C., L’Hoste, S., Offner, N., Picard, A., & Camonis, J. (2003). RLIP, an effector of the Ral GTPases, is a platform for Cdk1 to phosphorylate epsin during the switch off of endocytosis in mitosis. Journal of Biological Chemistry, 278(33), 30597–30604.
Sablina, A. A., Chen, W., Arroyo, J. D., Corral, L., Hector, M., Bulmer, S. E., Hahn, W. C., et al. (2007). The tumor suppressor PP2A Aβ regulates the RalA GTPase. Cell, 129(5), 969–982.
Sasaki, A. T., Carracedo, A., Locasale, J. W., Anastasiou, D., Takeuchi, K., Kahoud, E. R., Cantley, L. C., et al. (2011). Ubiquitination of K-Ras enhances activation and facilitates binding to select downstream effectors. Science Signaling, 4(163), ra14–ra15.
Seguin, L., Kato, S., Franovic, A., Camargo, M. F., Lesperance, J., Elliott, K. C., Husain, H., et al. (2014). An integrin β 3–KRAS–RalB complex drives tumour stemness and resistance to EGFR inhibition. Nature Cell Biology, 16(5), 457–468.
Seibold, M., Stühmer, T., Kremer, N., Mottok, A., Scholz, C.-J., Schlosser, A., Barrio, S., et al. (2020). RAL GTPases mediate multiple myeloma cell survival and are activated independently of oncogenic RAS. Haematologica, 105(9), 2316.
Shao, H., & Andres, D. A. (2000). A novel RalGEF-like protein, RGL3, as a candidate effector for rit and Ras. Journal of Biological Chemistry, 275(35), 26914–26924.
Shipitsin, M., & Feig, L. A. (2004). RalA but not RalB enhances polarized delivery of membrane proteins to the basolateral surface of epithelial cells. Molecular and Cellular Biology, 24(13), 5746–5756.
Shirakawa, R., Fukai, S., Kawato, M., Higashi, T., Kondo, H., Ikeda, T., Kimura, T., et al. (2009). Tuberous sclerosis tumor suppressor complex-like complexes act as GTPase-activating proteins for Ral GTPases. Journal of Biological Chemistry, 284(32), 21580–21588.
Shirakawa, R., & Horiuchi, H. (2015). Ral GTPases: Crucial mediators of exocytosis and tumourigenesis. The Journal of Biochemistry, 157(5), 285–299.
Shukla, S., Allam, U. S., Ahsan, A., Chen, G., Krishnamurthy, P. M., Marsh, K., Schipper, M., et al. (2014). KRAS protein stability is regulated through SMURF2: UBCH5 complex-mediated β-TrCP1 degradation. Neoplasia, 16(2), 115-W115.
Sidhu, R. S., Clough, R. R., & Bhullar, R. P. (2003). Ca2+/calmodulin binds and dissociates K-RasB from membrane. Biochemical and Biophysical Research Communications, 304(4), 655–660.
Siegel-Lakhai, W., Crul, M., Zhang, S., Sparidans, R., Pluim, D., Howes, A., Schellens, J., et al. (2005). Phase I and pharmacological study of the farnesyltransferase inhibitor tipifarnib (Zarnestra®, R115777) in combination with gemcitabine and cisplatin in patients with advanced solid tumours. British Journal of Cancer, 93(11), 1222–1229.
Simicek, M., Lievens, S., Laga, M., Guzenko, D., Aushev, V. N., Kalev, P., Tavernier, J., et al. (2013). The deubiquitylase USP33 discriminates between RALB functions in autophagy and innate immune response. Nature Cell Biology, 15(10), 1220–1230.
Sjogren, A.-K.M., Andersson, K. M., Liu, M., Cutts, B. A., Karlsson, C., Wahlstrom, A. M., Tarkowski, A., et al. (2007). GGTase-I deficiency reduces tumor formation and improves survival in mice with K-RAS–induced lung cancer. The Journal of Clinical Investigation, 117(5), 1294–1304.
Song, X., Hua, L., Xu, Y., Fang, Z., Wang, Y., Gao, J., Yu, R., et al. (2015). Involvement of RalB in the effect of geranylgeranyltransferase I on glioma cell migration and invasion. Clinical and Translational Oncology, 17(6), 477–485.
Sparano, J. A., Moulder, S., Kazi, A., Coppola, D., Negassa, A., Vahdat, L., Munster, P., et al. (2009). Phase II trial of tipifarnib plus neoadjuvant doxorubicin-cyclophosphamide in patients with clinical stage IIB-IIIC breast cancer. Clinical Cancer Research, 15(8), 2942–2948.
Sparano, J. A., Moulder, S., Kazi, A., Vahdat, L., Li, T., Pellegrino, C., Hoschander, S., et al. (2006). Targeted inhibition of farnesyltransferase in locally advanced breast cancer: A phase I and II trial of tipifarnib plus dose-dense doxorubicin and cyclophosphamide. Journal of Clinical Oncology, 24(19), 3013–3018.
Sugihara, K., Asano, S., Tanaka, K., Iwamatsu, A., Okawa, K., & Ohta, Y. (2002). The exocyst complex binds the small GTPase RalA to mediate filopodia formation. Nature Cell Biology, 4(1), 73–78.
Sun, J., Qian, Y., Hamilton, A. D., & Sebti, S. M. (1995). Ras CAAX peptidomimetic FTI 276 selectively blocks tumor growth in nude mice of a human lung carcinoma with K-Ras mutation and p53 deletion. Cancer Research, 55(19), 4243–4247.
Switzer, C. H., Cheng, R. Y., Ridnour, L. A., Glynn, S. A., Ambs, S., & Wink, D. A. (2012). Ets-1 is a transcriptional mediator of oncogenic nitric oxide signaling in estrogen receptor-negative breast cancer. Breast Cancer Research, 14(5), 1–13.
Tang, C.-H., Wei, W., & Liu, L. (2012). Regulation of DNA repair by S-nitrosylation. Biochimica Et Biophysica Acta (BBA)-General Subjects, 1820(6), 730–735.
Urano, T., Emkey, R., & Feig, L. A. (1996). Ral-GTPases mediate a distinct downstream signaling pathway from Ras that facilitates cellular transformation. The EMBO Journal, 15(4), 810–816.
Vigil, D., Martin, T. D., Williams, F., Yeh, J. J., Campbell, S. L., & Der, C. J. (2010). Aberrant overexpression of the Rgl2 Ral small GTPase-specific guanine nucleotide exchange factor promotes pancreatic cancer growth through Ral-dependent and Ral-independent mechanisms. Journal of Biological Chemistry, 285(45), 34729–34740.
Wahlstrom, A. M., Cutts, B. A., Liu, M., Lindskog, A., Karlsson, C., Sjogren, A.-K.M., Bergo, M. O., et al. (2008). Inactivating Icmt ameliorates K-RAS–induced myeloproliferative disease. Blood, the Journal of the American Society of Hematology, 112(4), 1357–1365.
Wang, C., Yuan, P., Xu, B., Yuan, L., Yang, H., & Liu, X. (2015). RLIP76 expression as a prognostic marker of breast cancer. European Review for Medical and Pharmacological Sciences, 19(11), 2105–2111.
Wang, H., Owens, C., Chandra, N., Conaway, M. R., Brautigan, D. L., & Theodorescu, D. (2010). Phosphorylation of RalB is important for bladder cancer cell growth and metastasis. Cancer Research, 70(21), 8760–8769.
Wang, M., Hossain, M., Tan, W., Coolman, B., Zhou, J., Liu, S., & Casey, P. (2010). Inhibition of isoprenylcysteine carboxylmethyltransferase induces autophagic-dependent apoptosis and impairs tumor growth. Oncogene, 29(35), 4959–4970.
Wang, Q., Wang, J.-Y., Zhang, X.-P., Lv, Z.-W., Fu, D., Lu, Y.-C., Chen, J.-X., et al. (2013). RLIP76 is overexpressed in human glioblastomas and is required for proliferation, tumorigenesis and suppression of apoptosis. Carcinogenesis, 34(4), 916–926.
White, M. A., Vale, T., Camonis, J. H., Schaefer, E., & Wigler, M. H. (1996). A role for the Ral guanine nucleotide dissociation stimulator in mediating Ras-induced transformation. Journal of Biological Chemistry, 271(28), 16439–16442.
Whyte, D. B., Kirschmeier, P., Hockenberry, T. N., Nunez-Oliva, I., James, L., Catino, J. J., Pai, J.-K., et al. (1997). K-and N-Ras are geranylgeranylated in cells treated with farnesyl protein transferase inhibitors. Journal of Biological Chemistry, 272(22), 14459–14464.
Williams, J. G., Pappu, K., & Campbell, S. L. (2003). Structural and biochemical studies of p21Ras S-nitrosylation and nitric oxide-mediated guanine nucleotide exchange. Proceedings of the National Academy of Sciences, 100(11), 6376–6381.
Willumsen, B. M., Christensen, A., Hubbert, N. L., Papageorge, A. G., & Lowy, D. R. (1984). The p21 ras C-terminus is required for transformation and membrane association. Nature, 310(5978), 583–586.
Winter-Vann, A. M., Kamen, B. A., Bergo, M. O., Young, S. G., Melnyk, S., James, S. J., & Casey, P. J. (2003). Targeting Ras signaling through inhibition of carboxyl methylation: An unexpected property of methotrexate. Proceedings of the National Academy of Sciences, 100(11), 6529–6534.
Wolda, S. L., & Glomset, J. (1988). Evidence for modification of lamin B by a product of mevalonic acid. Journal of Biological Chemistry, 263(13), 5997–6000.
Wu, Z., Owens, C., Chandra, N., Popovic, K., Conaway, M., & Theodorescu, D. (2010). RalBP1 is necessary for metastasis of human cancer cell lines. Neoplasia, 12(12), 1003–1012.
Xia, S., Forman, L. W., & Faller, D. V. (2007). Protein kinase Cδ is required for survival of cells expressing activated p21RAS. Journal of Biological Chemistry, 282(18), 13199–13210.
Xu, L., Lubkov, V., Taylor, L. J., & Bar-Sagi, D. (2010). Feedback regulation of Ras signaling by Rabex-5-mediated ubiquitination. Current Biology, 20(15), 1372–1377.
Yan, C., Liu, D., Li, L., Wempe, M. F., Guin, S., Khanna, M., Wysoczynski, C. L., et al. (2014). Discovery and characterization of small molecules that target the GTPase Ral. Nature, 515(7527), 443–447.
Yan, C., & Theodorescu, D. (2018). RAL GTPases: Biology and potential as therapeutic targets in cancer. Pharmacological Reviews, 70(1), 1–11.
Yan, H., Jahanshahi, M., Horvath, E. A., Liu, H.-Y., & Pfleger, C. M. (2010). Rabex-5 ubiquitin ligase activity restricts Ras signaling to establish pathway homeostasis in Drosophila. Current Biology, 20(15), 1378–1382.
Yang, M. H., Laurent, G., Bause, A. S., Spang, R., German, N., Haigis, M. C., & Haigis, K. M. (2013). HDAC6 and SIRT2 regulate the acetylation state and oncogenic activity of mutant K-RAS. Molecular Cancer Research, 11(9), 1072–1077.
Yang, M. H., Nickerson, S., Kim, E. T., Liot, C., Laurent, G., Spang, R., Bar-Sagi, D., et al. (2012). Regulation of RAS oncogenicity by acetylation. Proceedings of the National Academy of Sciences, 109(27), 10843–10848.
Yang, Q., Lang, C., Wu, Z., Dai, Y., He, S., Guo, W., Peng, X., et al. (2019). MAZ promotes prostate cancer bone metastasis through transcriptionally activating the KRas-dependent RalGEFs pathway. Journal of Experimental & Clinical Cancer Research, 38(1), 1–17.
Zago, G., Veith, I., Singh, M. K., Fuhrmann, L., De Beco, S., Remorino, A., Brandon, N., et al. (2018). RalB directly triggers invasion downstream Ras by mobilizing the Wave complex. eLife, 7, e4074.
Zhu, Z., Aref, A. R., Cohoon, T. J., Barbie, T. U., Imamura, Y., Yang, S., Thai, T. C., et al. (2014). Inhibition of KRAS-driven tumorigenicity by interruption of an autocrine cytokine circuit. Cancer Discovery, 4(4), 452–465.
Zinatizadeh, M. R., Miri, S. R., Zarandi, P. K., Chalbatani, G. M., Raposo, C., Mirzaei, H. R., Mahmoodzadeh, H., et al. (2021). The Hippo Tumor Suppressor Pathway (YAP/TAZ/TEAD/MST/LATS) and EGFR-RAS-RAF-MEK in cancer metastasis. Genes Dis, 8(1), 48–60. https://doi.org/10.1016/j.gendis.2019.11.003
Zinatizadeh, M. R., Momeni, S. A., Zarandi, P. K., Chalbatani, G. M., Dana, H., Mirzaei, H. R., Miri, S. R., et al. (2019). The role and function of Ras-association domain family in cancer: a review. Genes & Diseases, 6(4), 378–384.
Zipfel, P. A., Brady, D. C., Kashatus, D. F., Ancrile, B. D., Tyler, D. S., & Counter, C. M. (2010). Ral activation promotes melanomagenesis. Oncogene, 29(34), 4859–4864.
Author information
Affiliations
Department of Biology, Science and Research Branch, Islamic Azad University, Tehran, Iran
Mohammad Reza Zinatizadeh & Peyman Kheirandish Zarandi
Cancer Biology Signaling Pathway Interest Group (CBSPIG), Universal Scientific Education and Research Network (USERN), Tehran, Iran
Mohammad Reza Zinatizadeh & Peyman Kheirandish Zarandi
School of Medicine, Tehran University of Medical Sciences, Tehran, Iran
Mahsa Keshavarz-Fathi
Cancer Immunology Project (CIP), Universal Scientific Education and Research Network (USERN), Tehran, Iran
Mahsa Keshavarz-Fathi
Department of Mycobacteriology and Pulmonary Research, Pasteur Institute of Iran, Tehran, Iran
Mohammad Hadi Yousefi
Department of Mycobacteriology and Pulmonary Research, Microbiology Research Center (MRC), Pasteur Institute of Iran, Tehran, Iran
Mohammad Hadi Yousefi
Research Center for Immunodeficiencies, Children’s Medical Center, Tehran University of Medical Sciences, Tehran, Iran
Nima Rezaei
Department of Immunology, School of Medicine, Tehran University of Medical Sciences, Tehran, Iran
Nima Rezaei
Network of Immunity in Infection, Malignancy and Autoimmunity (NIIMA), Universal Scientific Education and Research Network (USERN), Tehran, Iran
Nima Rezaei
Corresponding author
Correspondence to Mohammad Reza Zinatizadeh.
Ethics declarations
Conflict of interest
The authors report no conflicts of interest in this work.
Rights and permissions
About this article
Cite this article
Zinatizadeh, M.R., Zarandi, P.K., Keshavarz-Fathi, M. et al. The role of ral signaling and post translational modifications (PTMs) of Ras in cancer. GENOME INSTAB. DIS. 3, 22–32 (2022). https://doi.org/10.1007/s42764-022-00059-0
Received02 November 2021
Revised26 December 2021
Accepted06 January 2022
Published17 January 2022
Issue DateFebruary 2022
DOIhttps://doi.org/10.1007/s42764-022-00059-0
Share this article
Anyone you share the following link with will be able to read this content:
Get shareable linkKeywords
Cancer
Ras proteins
Ral effectors
Signaling
Post translational modifications
用户登录
还没有账号?
立即注册