New insights into the mechanism of RPA in preserving genome stability
Review Article
Simin Zhang, Xuejie Wang, Han Zhao, Jingyao Shi & Xuefeng Chen
Genome Instability & Disease , 3 255–266 (2022)
Abstract
Single-stranded DNA (ssDNA) is a common intermediate produced during normal DNA transactions, including transcription, DNA replication, repair, and recombination. Replication protein A (RPA), an evolutionarily conserved ssDNA-binding protein complex, plays a critical role in these DNA transactions through its dynamic association with ssDNA or proteins. The binding of RPA on ssDNA protects ssDNA from unscheduled nuclease digestion, melts secondary DNA structures, suppresses mutations, and signals the checkpoint for DNA damage or replication stresses. In parallel, RPA serves as a platform for interacting with a number of different proteins to coordinate various DNA metabolic processes. However, our understanding of the regulation and function of RPA is far from complete. Here, we reviewed recent advances on the roles of RPA in regulating replication, repair, nucleosome assembly, rDNA transcription, chromatin epigenetic landscape, and chromosome segregation. In addition, we described the interplay between RPA and the ubiquitin E3 ligase RFWD3 that determines RPA turnover at replication forks or DNA lesion sites. These new findings advance our understanding of the versatile roles of RPA in preserving genome integrity and could provide opportunities to develop therapeutic strategies targeting cancer.
An overview of RPA
To maintain a normal cell life cycle, the eukaryotic genome undergoes active DNA metabolisms, including transcription, replication, and repair. DNA transactions during these fundamental nuclear processes cause the exposure of single-stranded DNA (ssDNA), one of the most ubiquitous and important biological intermediates. However, ssDNA is intrinsically vulnerable to nuclease attack and tends to form secondary structures that can impede normal DNA metabolism (Chen & Wold, 2014). Therefore, cells have evolved various ssDNA-binding proteins (SSBs) that protect ssDNA and assist ssDNA-associated DNA transactions.
The first example of SSB proteins, the bacteriophage T4 gene 32 protein, was discovered and characterized by Alberts and Frey (1970). This protein functions in DNA replication and recombination by catalyzing DNA denaturation and renaturation (Alberts & Frey, 1970). Soon after, other prokaryotic SSBs that are known to function in DNA metabolism have been identified, such as the E. coli SSB protein (Eco SSB) (de Vries & Wackernagel, 1993; Lohman & Ferrari, 1994; Sigal et al., 1972). Eco SSB stimulates the activity of E. coli DNA polymerase II and pol III holoenzyme and facilitates several processes mediated by the recombinase RecA (Fay et al., 1982; Kowalczykowski, 1991; Molineux et al., 1974; Roca & Cox, 1990). Replication Protein A (RPA), the primary SSB protein in eukaryotes, was originally purified from human cell extracts by three independent groups and was found to be an essential component of the simian virus 40 replication system (Fairman & Stillman, 1988; Wobbe et al., 1987; Wold & Kelly, 1988). Shortly, Brill and Stillman found the evolutionarily conserved protein complex of RPA in Saccharomyces cerevisiae (Brill & Stillman, 1989, 1991).
The ssDNA-binding protein complex RPA participates in nearly all aspects of DNA metabolism (Bae et al., 2001; Caldwell & Spies, 2020; Dornreiter et al., 1992; Haber, 2016; Kowalczykowski, 2015; Li, 2008; Symington et al., 2014; Waga & Stillman, 1994; Yuzhakov et al., 1999). Due to its high affinity (up to 10–10 M) to ssDNA, RPA is thought to be the first responder for ssDNA (Bhat & Cortez, 2018; Chen & Wold, 2014). RPA is a heterotrimer complex composed of three subunits, i.e., Rfa1, Rfa2, and Rfa3 in yeast or RPA70, RPA32, and RPA14 in human. The RPA complex contains a total of six oligonucleotide/oligosaccharide-binding (DNA-binding domains, DBD) motifs, four in the Rfa1 subunit (DBD-A, DBD-B, DBD-C, and DBD-F), and one each in Rfa2 (DBD-D) and Rfa3 (DBD-E) (Fig. 1; Caldwell & Spies, 2020; Chen & Wold, 2014; Marechal & Zou, 2015). These motifs mediate the dynamic association of RPA with ssDNA or other proteins (Chen & Wold, 2014; Iftode et al., 1999; Wold, 1997). Among these motifs, the DBD-A, DBD-B, and DBD-F of RPA70 and the winged-helix (WH) domain of RPA32 are the primary protein–protein interaction surfaces (Caldwell & Spies, 2020). Given the broad functions of RPA in DNA metabolism, a number of RPA-interacting proteins have been uncovered (Caldwell & Spies, 2020; Fanning et al., 2006; Marechal & Zou, 2015; Zou et al., 2006). RPA can bind short (8–10 nt) or long (28–30 nt) ssDNA in different modes and diffuse within the bound DNA ligand (Arunkumar et al., 2003; Bhat & Cortez, 2018; Deng et al., 2014; Fan & Pavletich, 2012; Gibb et al., 2014; Marnef et al., 2017; Pokhrel et al., 2019). The cellular functions of RPA rely on its high affinity to ssDNA and its ability to interact with different proteins (Caldwell & Spies, 2020; Pokhrel et al., 2019).
Fig. 1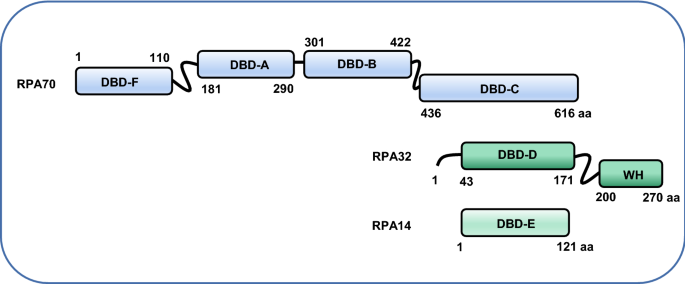
Schematic showing the three subunits of the human RPA complex. RPA70, RPA32, and RPA14 are indicated in different colors. The DBD-C, -D, and -E mediate the assembly of the RPA complex. The border of each DBD domain is indicated. aa, amino acid
Full size imagePrevious studies have revealed the key functions of RPA in protecting ssDNA, removing secondary structures, inhibiting mutations, recruiting other proteins, and in signaling the DNA damage checkpoint (Acharya et al., 2021; Chen & Wold, 2014; Zou & Elledge, 2003). Therefore, RPA is essential for all fundamental nuclear processes, including DNA replication, repair, and recombination, and is crucial for preventing chromosome catastrophe (Caldwell & Spies, 2020; Chen & Wold, 2014; Marechal & Zou, 2015; Toledo et al., 2013; Wold, 1997). These functions are documented in many excellent reviews (Byrne & Oakley, 2019; Caldwell & Spies, 2020; Chen & Wold, 2014; Iftode et al., 1999; Krasikova et al., 2016; Marechal & Zou, 2015; Wold, 1997). In this review, we will focus on the recent progress of RPA in regulating replication, repair, nucleosome assembly, rDNA transcription, chromatin epigenetic landscape, and chromosome segregation. Finally, we will also discuss the ubiquitination and turnover of RPA on chromatin.
RPA delivers ssDNA to the nuclease Dna2
RPA interacts with many different enzymes or factors to coordinate DNA transactions or signal the ssDNA intermediate (Caldwell & Spies, 2020). Dna2 is a conserved nuclease–helicase functioning in DNA replication and recombination (Zheng et al., 2020). It removes the RPA-coated long 5′-flap structure and allows Fen1 nuclease to further complete lagging strand maturation (Bae et al., 2001; Zheng et al., 2020). At DNA double-stranded break (DSB) ends, Dna2 cleaves the RPA-coated 5′-strand unwound by the Sgs1 helicase (Zhu et al., 2008). RPA interacts with Dna2, and this interaction stimulates the recruitment and the nuclease and helicase activities of Dna2 (Cejka et al., 2010; Zheng et al., 2020). As a result, disruption of their interaction inhibits the nuclease activity of Dna2 (Niu et al., 2010).
Recently, Shen et al. proposed a gating mechanism, where RPA can act as an active unit to deliver ssDNA to Dna2 nuclease (Shen et al., 2022). The authors found that RPA is dispensable for Dna2-mediated ssDNA digestion under low concentrations of salts but becomes essential under higher salt conditions, implying that RPA plays additional functions in facilitating Dna2 cleavage besides its role in promoting Dna2 recruitment (Shen et al., 2022). Using DNA curtains and electrophoretic mobility shift assays (EMSA), Shen and colleagues discovered that Dna2 becomes a processive enzyme only after being ensembled with RPA to form the Dna2–RPA–ssDNA ternary complex. Notably, the fusion of the N-terminal DBD-A/B of Rfa1 to Dna2 lacking the N-terminal end (Dna2-Δ248N), which forces Rfa1 and Dna2 to function as a single unit, stimulates the initial cleavage and progressivity of Dna2 nuclease, yet it fails to overcome secondary DNA structures (Shen et al., 2022). Based on this observation, the authors reasoned that RPA might play a role in delivering ssDNA to Dna2. Since Dna2 interacts with the N-terminal end of Rfa1, they speculated that there should be a gate at the N-terminus of RPA that mediates DNA transportation to Dna2 (Shen et al., 2022). Indeed, they identified that the residue Y193 of Rfa1 is critical to support the initial 5′-cleavage and the processivity of Dna2 (Shen et al., 2022). Therefore, the authors proposed that the linker region between DBD-F and DBD-A may play a role in the gating of ssDNA. Thus, RPA facilitates Dna2-mediated ssDNA digestion by recruiting the enzyme, eliminating DNA secondary structures, and delivering ssDNA to Dna2 (Shen et al., 2022). This mechanism may also apply to other nucleases or helicases regulated by RPA.
RPA cooperates with Rad54 to promote homology search
As the major ssDNA sensor, RPA directly participates in multiple steps during DSB repair by homologous recombination (HR). Once ssDNA is generated by DNA end resection, RPA rapidly binds to the 3′-end ssDNA. In yeast cells, RPA was reported to play a role in protecting the 3′-end ssDNA (Chen et al., 2013; Ruff et al., 2016), yet this role seems to be weak in vitro (Niu et al., 2010). Notably, a recent study in human cells showed that the 3′-end ssDNA is protected by the transient formation of RNA–DNA hybrids transcribed by RNA polymerase III at DNA breaks (Liu & Kong, 2021; Liu et al., 2021b). Subsequently, Rad51 recombinase replaces the ssDNA-bound RPA to form the presynaptic complex (PSC) that drives the search for a homologous donor template, forming a D-loop intermediate (Mehta et al., 2017; Nimonkar et al., 2011). However, the detailed mechanism underlying homology recognition remains elusive.
The emergence of high-throughput single-molecule imaging techniques makes it possible to visibly monitor the behaviors of DNA repair or recombination proteins at the single-molecule level (Gorman et al., 2007; Greene et al., 2010; Prasad et al., 2007; Visnapuu & Greene, 2009). It was suggested that homology search involves a combination of three-dimensional intersegmental transfer, short-distance one-dimensional diffusion, and length-dependent homology recognition (Crickard et al., 2020). Rad54, a member of the Swi2/Snf2 family of DNA motor protein, is a key HR repair factor. Rad54 can promote strand invasion, catalyze nucleosome remodeling, and remove Rad51 from dsDNA (Alexeev et al., 2003; Amitani et al., 2006; Ceballos & Heyer, 2011; Petukhova et al., 1998; Ristic et al., 2001; Wright & Heyer, 2014; Zhang et al., 2007). Using the DNA curtain technique, Crickard and colleagues recently observed that Rad54 drives PSC translocation along donor DNA and can switch the homology search from the diffusion-based pathways to an active process in which DNA sequences are aligned via an ATP-dependent molecular motor-driven mechanism (Crickard et al., 2020). Notably, the authors observed that RPA co-localizes with Rad54 on the PSC and tracks with the PSC in a manner dependent on the ATPase activity of Rad54 (Crickard et al., 2020). The absence of RPA causes a very significant reduction (~ 47% for 21-nt tailed duplex) in overall first-passage recognition efficiency and results in an even more dramatic defect (~ 65% for 21-nt tailed duplex) when the PSC approached from the incorrect direction (Crickard et al., 2020). The uneven impact caused by the absence of RPA suggests that RPA plays a role in accelerating the homology search by allowing the PSC to concurrently sample both strands of the donor dsDNA for homology regardless of strand polarity (Crickard et al., 2020). Collectively, these results led to the conclusion that RPA cooperates with Rad54 to enable bidirectional homology sampling. Although the detailed mechanism is unclear, it was hypothesized that the ssDNA bound by RPA might bend into a U-shaped configuration, where the polarity of one-half of the ssDNA is reversed and can be recognized by the PSC (Crickard et al., 2020).
Dynamic binding of RPA on ssDNA promotes high-fidelity DNA replication and repair
Faithful DNA replication and repair are essential for the maintenance of genome stability. RPA depletion, haploinsufficiency, or exhaustion can lead to spontaneous mutations, DNA replication catastrophe, misusage of repair pathways, and genome instability (Chen et al., 1998; Hass et al., 2010; Schramke et al., 2004; Toledo et al., 2013). Recent studies showed that dynamic regulation of RPA binding on ssDNA is important to promote high-fidelity DNA replication and repair (Deng et al., 2014; Li et al., 2018; Wang et al., 2021).
Besides the HR and non-homologous end joining (NHEJ) pathways, DSBs can also be repaired by a backup mechanism termed microhomology-mediated end joining (MMEJ). It repairs DSBs via annealing of microhomologies exposed by DNA end resection in a Ku- and ligase IV-independent manner and generates mutagenic repair products losing the sequence between the repeats (McVey & Lee, 2008). Deng et al. found that MMEJ is barely used (0.006%) to repair DSBs in wild-type yeast cells. However, rfa1-t48, a hypomorphic RFA1 mutation perturbing the interaction between RPA and ssDNA, increases the frequency of MMEJ up to 350-fold (Deng et al., 2014). Therefore, the authors proposed that RPA plays a role in channeling ssDNA intermediates from MMEJ to error-free HR, likely by preventing annealing between short homologies (Deng et al., 2014; Sugiyama et al., 1998).
This conclusion is further strengthened by our recent studies (Wang et al., 2021). Rtt105, a protein initially identified as a regulator of the Ty1 retrotransposon, functions as the chaperone protein for RPA (Li et al., 2018). Li et al. found that Rtt105 facilitates DNA replication by mediating the nuclear import of RPA and stimulating RPA binding to ssDNA (Fig. 2; Li et al., 2018). Interestingly, Wang et al. recently found that Rtt105 promotes high-fidelity DNA replication and repair via regulating dynamic RPA binding on ssDNA (Wang et al., 2021). The loss of Rtt105 causes increased spontaneous mutations featured with micro-homology-mediated large insertions or deletion, and these events appear to relate to polymerase slippage or the repair by single-strand annealing (SSA) (Wang et al., 2021). In rtt105Δ cells, DSB repair by the error-free gene conversion is attenuated, while the repair by the mutagenic MMEJ or SSA pathway is increased (Fig. 2). Thus, Rtt105 promotes accurate HR while suppressing the deleterious repair pathways. These defects were not due to the altered RPA nuclear localization in rtt105Δ cells. Instead, Wang et al. showed that Rtt105 is recruited to DSB ends in vivo. Using biochemical and single-molecule approaches, the authors demonstrated that Rtt105 directly stimulates the dynamic binding of RPA on ssDNA in vitro (Wang et al., 2021). Previous studies by Li et al. and our work revealed that the two residues E171 and L172 on Rtt105 and the single residue V106 on Rfa1 are critical to mediate the interaction between Rtt105 and RPA (Li et al., 2018; Wang et al., 2021). Using the rtt105-EL2A or rfa1-V106A point mutant, the authors demonstrated that specific disruption of the Rtt105–RPA interaction causes similar phenotypes as seen in rtt105Δ null cells, including increased mutations, large insertions/deletions, attenuated gene conversion and elevated SSA (Wang et al., 2021). Thus, the authors concluded that Rtt105-mediated regulation of RPA is important for faithful DNA replication and repair (Wang et al., 2021).
Fig. 2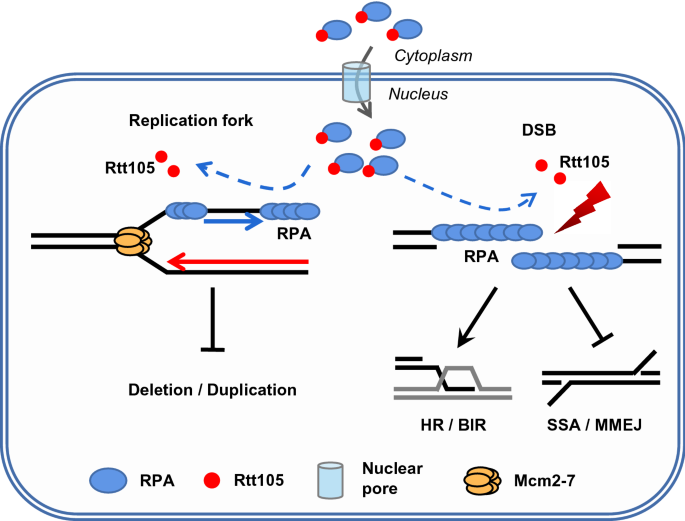
Working model for the role of Rtt105 in promoting high-fidelity DNA replication and repair via regulating RPA. Rtt105 regulates the nuclear import of RPA and promotes RPA loading at replication forks or DNA breaks via their physical interaction. Rtt105-mediated regulation of RPA promotes high-fidelity gene conversion or BIR while suppressing mutagenic replication and deleterious repair by SSA or MMEJ
Full size imageRPA defines the chromatin epigenetic landscape
Eukaryotic cells have evolved a complex signaling network that integrates a plethora of protein post-translational modifications to preserve genome stability. A central player among these events is the mono-ubiquitination of histone H2B on K123 in yeast or K120 in mammals (H2Bub). This modification is catalyzed by the E2 ubiquitin conjugase Rad6 (Ubc6 or RAD6 in human) in cooperation with the E3 ubiquitin ligase Bre1 (RNF20/RNF40 in mammals) (Hwang et al., 2003; Robzyk et al., 2000; Sun & Allis, 2002; Wood et al., 2003; Zhu et al., 2005). H2Bub plays a role in regulating chromatin structure and nucleosome dynamics, thereby allowing the access of DNA by protein machinery (Fierz et al., 2011; Moyal et al., 2011; Nakamura et al., 2011; Trujillo & Osley, 2012; Zheng et al., 2018). As a result, H2Bub is important for proper DNA replication, repair, and transcription across species (Chernikova et al., 2010, 2012; Fuchs & Oren, 2014; Hung et al., 2017; Moyal et al., 2011; Nakamura et al., 2011; Northam & Trujillo, 2016; Robzyk et al., 2000; Trujillo & Osley, 2012; Wood et al., 2003; Zeng et al., 2016; Zhu et al., 2005). Furthermore, the ligase Bre1 or its human homolog RNF20/RNF40 has been shown to be recruited to replication forks or DNA breaks to stimulate local H2Bub (Moyal et al., 2011; Nakamura et al., 2011; Zheng et al., 2018). However, how the Bre1–H2Bub pathway is evoked to respond to the DNA replication and repair was unknown.
Recently, Liu et al. found that Bre1 is recruited by the RPA–ssDNA complex to DNA breaks or replication forks (Fig. 3; Liu et al., 2021a). The authors showed that RPA physically interacts with Bre1 in vitro and in vivo, and the interaction is stimulated by ssDNA. Using pull-down and immunoprecipitation assays, Liu et al. identified six residues (L516, L518, D520, D522, L524, and L525) in Bre1 and the single residue D465 in Rfa1 are critical to mediate the RPA–Bre1 interaction. Mutation of the six residues in Bre1(bre1-6A) or the single residue in Rfa1(rfa1-D465A) to alanine ablates the interaction. Using these point mutants, the authors demonstrated that specific disruption of the Bre1–RPA interaction impairs the enrichment of Bre1 and H2Bub at replication forks or DSB ends, leading to defects in DNA replication, response to replication stress, and repair by HR (Fig. 3). However, the point mutation bre1-6A or rfa1-D465A did not affect the E3 ligase activity of Bre1 or the ssDNA binding ability of Rfa1. These results led to the conclusion that RPA couples H2Bub to DNA replication and repair through physically recruiting the E3 ligase Bre1 to chromatin. In other words, RPA defines the chromatin H2Bub landscape that ensures proper replication and repair (Liu et al., 2021a).
Fig. 3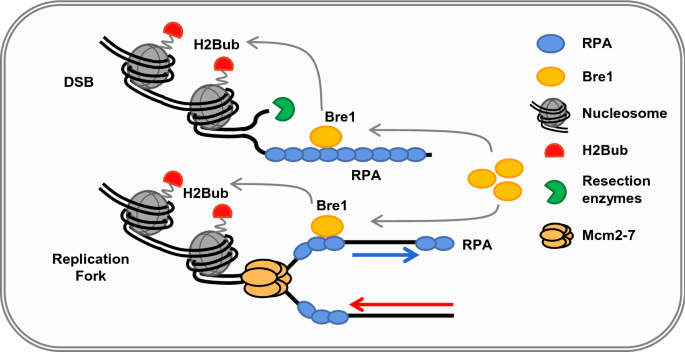
RPA defines H2Bub chromatin landscape during DNA replication and repair. RPA directly recruits Bre1 to replication forks or DSBs via their physical interaction. The enrichment of Bre1 at replications forks or DSBs stimulates local H2Bub, thereby promoting DNA replication, response to the replication stress, and repair by HR
Full size imageAnother work in fission yeast also implied the relationship between RPA–ssDNA and H2Bub (Zeng et al., 2016). The authors showed that Rhp6, the homolog of Rad6 in fission yeast, interacts with RPA, and this interaction facilitates the recruitment of Rhp6 to the initial resection sites to form a core H2Bub domain (Zeng et al., 2016). Intriguingly, this initial core domain is vital for the subsequent Wdr70-dependent distal spreading of H2Bub, which may facilitate long-range resection in an Exo1-dependent manner (Zeng et al., 2016). Notably, Liu et al. also noted that RNF20, the human homolog of yeast Bre1, interacts with RPA via the evolutionarily conserved motifs, suggesting that the role of RPA in defining H2Bub chromatin landscape is likely common across species (Liu et al., 2021a).
RPA promotes replication-coupled nucleosome assembly
DNA replication-coupled nucleosome assembly is essential to maintain genome integrity and epigenetic information. Previous work showed that multiple histone chaperones assist the deposition of histones on newly synthesized DNA (Gurard-Levin et al., 2014; Huang et al., 2005; Smith & Stillman, 1989; Tyler et al., 1999; VanDemark et al., 2006; Yang et al., 2016). However, how nucleosome deposition is coupled to DNA replication was unclear. During replication, RPA binds ssDNA unwound by the replicative helicase MCM to ensure the movement of the replisome (Brill & Stillman, 1989). Importantly, Liu et al. recently identified that RPA plays a key role in replication-coupled nucleosome assembly (Liu et al., 2017).
The authors found that RPA directly interacts with free histones H3–H4 in vivo and in vitro, and this interaction is enhanced in the presence of ssDNA, suggesting that this interaction occurs on chromatin (Liu et al., 2017). Further studies showed that the RPA–H3–H4 interaction peaks in the S phase and the complex contains acetylated histones H3K56Ac, H4K5Ac, and H4K12Ac, markers of newly synthesized histones. Interestingly, in cells lacking H3K56Ac or chaperones involved in replication-coupled nucleosome assembly, the RPA–H3–H4 interaction is decreased, implying that the RPA–H3–H4 interaction occurs concurrently with nucleosome assembly (Liu et al., 2017). Using biochemical experiments, the authors provided direct evidence that RPA residing on ssDNA binds free H3–H4 to promote their assembly onto adjacent dsDNA (Liu et al., 2017). To examine nucleosome formation on nascent chromatin, they developed the replication-intermediate nucleosome mapping (ReIN-Map) technique, which involves parallel analyses of the nucleosome formation using MNase digestion of chromatin (MNase-seq) and of the newly synthesized DNA levels using sonication shearing of chromatin (Sonication-seq) (Xu et al., 2021). The ReIN scores in rfa1-A88P, a mutant allele with reduced H3–H4 binding, are reduced surrounding autonomously replicating sequences (ACSs), although the overall organization of nucleosomes on the mature chromatin is not affected. Thus, disruption of the RPA–H3–H4 interaction impairs the nucleosome assembly on nascent chromatin (Liu et al., 2017). These results support a model in which RPA binds ssDNA during DNA synthesis to facilitate the nucleosome assembly on nascent chromatin by providing a binding platform for histone H3–H4 and promoting their deposition onto the adjacent dsDNA (Liu et al., 2017). Given that RPA also interacts with the histone chaperones Caf1, FACT, and Rtt106, the authors proposed that RPA provides a common binding platform to ensure proper histone deposition during replication-coupled nucleosome assembly (Liu et al., 2017).
RPA senses R-loops to prevent genome instability
R-loop is a three-stranded nucleic acid structure consisting of a DNA–RNA hybrid and a displaced non-template ssDNA (Crossley et al., 2019; Garcia-Muse & Aguilera, 2019; Hegazy et al., 2020; Niehrs & Luke, 2020). It is a common transcription intermediate occurring in the genome. R-loops play an important role in regulating gene expression, DNA replication, and epigenetic modifications (Crossley et al., 2019; Garcia-Muse & Aguilera, 2019; Hegazy et al., 2020; Niehrs & Luke, 2020). However, aberrant accumulation of R-loops can also lead to DNA damage and genome instability (Hegazy et al., 2020; Niehrs & Luke, 2020). R-loop has been linked to many human diseases, including cancer, neurological disorders, and autoimmune diseases (Crossley et al., 2019; Garcia-Muse & Aguilera, 2019; Hegazy et al., 2020; Niehrs & Luke, 2020). Thus, properly sensing and controlling R-loop dynamics is important for preventing genome instability.
RPA acts as a master sensor of R-loops arising from different resources (Nguyen et al., 2017). Nguyen et al. proposed that RPA bound on the ssDNA of R-loops promotes the removal of harmful R-loops and functions as a regulator of RNaseH1, an enzyme specifically cleaving the RNA in RNA:DNA hybrids (Fig. 4; Nguyen et al., 2017). The authors found that RPA interacts with RNaseH1 and colocalizes with RNaseH1 and R-loops in cells. Purified RPA promotes the cleavage of R-loops by RNaseH1 in a concentration-dependent manner by stimulating the binding and the activity of RHaseH1 on R-loops (Nguyen et al., 2017). However, RPA cannot efficiently stimulate the RPA binding-defective RNaseH1 mutant (RNaseH1R57A or RNaseH13RA) in vitro. Consistently, the mutant RNaseH1 fails to accumulate at R-loops in cells and loses the ability to suppress R-loops and associated genomic instability (Nguyen et al., 2017). Thus, the interaction between RPA and RNaseH1 is critical for eliminating the aberrant accumulation of R-loops in cells.
Fig. 4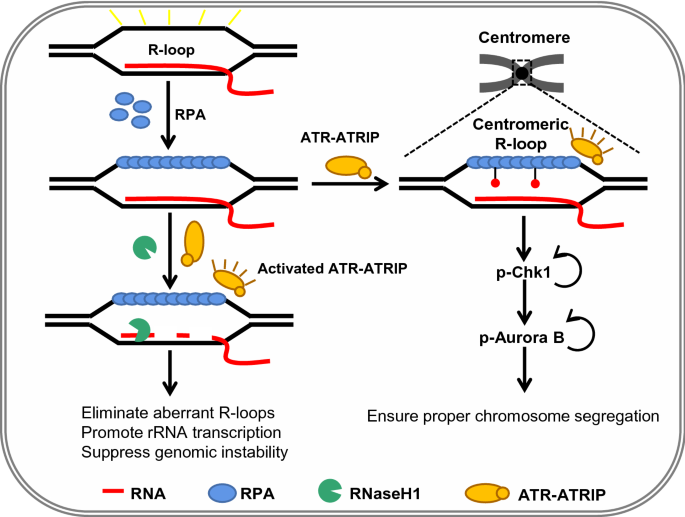
RPA acts as the sensor of R-loops to preserve genomic stability. RPA senses R-loops through binding the ssDNA of R-loops. On the one hand, the RPA bound on R-loops recruits RNaseH1 and stimulates its activity to prevent aberrant accumulation of R-loops. On the other hand, the RPA–ssDNA structure activates ATR, which is critical for suppressing genomic instability caused by the accumulation of R-loops. In addition, RPA binds nucleolar R-loops and regulates rRNA transcription and nucleolar structure. Finally, in mitosis, RPA binds to R-loops at centromeres and stimulates ATR activity to ensure proper chromosome segregation by activating downstream kinase Chk1 and Aurora B
Full size imageIn addition, RPA likely recruits the checkpoint proteins ATRIP–ATR to R-loops to activate the checkpoint, as noted for its role in the DNA damage response (Nguyen et al., 2018). It has been shown that perturbation of RNA splicing by expressing U2AF1S34F, a prevalent mutation of the splicing factor U2AF1 in cancers, causes accumulation of R-loops and the activation of ATR (Nguyen et al., 2018). Nguyen et al. noted that phosphorylated RPA (RPA32 pS33) colocalizes with R-loops, while suppression of R-loops by expressing RNaseH1 reduces the levels of phosphorylated RPA, suggesting that the R-loop structure itself causes the activation of ATR. Therefore, it was reasoned that RPA recruits the ATR–ATRIP complex to R-loops, which in turn phosphorylates RPA (Nguyen et al., 2018). Interestingly, inhibition of ATR induces a higher level of γ-H2AX in the splicing defective mutant cells, indicating that RPA-mediated ATR activation is essential to prevent extra DNA damage caused by the accumulation of R-loops (Matos et al., 2020; Nguyen et al., 2018). Thus, aberrant R-loop accumulation becomes a source of lethal DNA damage when ATR is inhibited, offering a potential strategy to therapeutically target the cells harboring splicing factor mutations (Matos et al., 2020; Nguyen et al., 2018).
RPA binds nucleolar R-loops and regulates rRNA transcription and nucleolar structure
Maintenance of nucleolar homeostasis is crucial for cells to respond to stresses and is typically linked to inhibition of rRNA transcription and changes in nucleolar morphology and composition (Feng & Manley, 2021; Grummt, 2013). Feng et al. recently reported the role of RPA in maintaining nucleolar homeostasis under conditions that lead to the formation of excess R-loops on rDNA (Fig. 4; Feng & Manley, 2021). The authors observed that depletion of SETX, a DNA:RNA helicase, or inhibition of topoisomerase I (Top1) results in accumulation of RPA in nucleolar foci and that this localization depends on the presence of R loops on rDNA as the expression of RNaseH1 significantly reduces the nucleolar localization of RPA (Feng & Manley, 2021). Consistently, RPA also colocalizes with RNA polymerase I in an R-loop-dependent manner. Notably, depletion of RPA results in increased levels of R-loops and γH2AX in the nucleolus and elevated levels of RNA Pol I occupancy on rDNA, suggesting a role of RPA in preventing excessive R-loops and associated DNA damage in the nucleolus. As a result, depletion of RPA leads to a significant decrease of the 47S pre-rRNA level when RNaseH1 is inactive (Feng & Manley, 2021). Strikingly, RPA depletion also causes the segregation and fragmentation of nucleolar structures into smaller sizes or reduced mass (Feng & Manley, 2021). Thus, RPA acts as a critical guardian of nucleolar structure and function and ensures proper rRNA transcription by a mechanism involving R-loop resolution.
RPA binds R-loops at centromeres to ensure proper chromosome segregation
Kabeche et al. recently found that the checkpoint kinase ATR localizes to centromeres, and this localization requires the interaction between ATR and the Aurora A-TPX2 complex or CENP-F (Kabeche et al., 2018). Inhibition of ATR increases the rate of lagging chromosomes in anaphase. It turns out to result from the reduced autophosphorylation of Aurora B kinase (p-Aurora B) in prometaphase after ATR inhibition. The result suggests that ATR is required for the full activation of Aurora B at centromeres (Kabeche et al., 2018). The authors then asked how ATR is activated at centromeres. Interestingly, they detected enrichment of R-loops and RPA at centromeres. Expression of wild-type RNaseH1 abolishes R-loop enrichment at centromeres, leading to impaired RPA and ATR localization, attenuated p-RPA and p-ATR staining, and reduced p-Aurora B at centromeres, accompanied by increased lagging chromosomes (Kabeche et al., 2018). These results indicate that the enrichment of R-loops and RPA at centromeres is critical for local ATR activation to ensure accurate chromosome segregation (Fig. 4; Kabeche et al., 2018). Thus, ATR is recruited to the vicinity of centromeres via association with Aurora A and CENP-F in mitosis, while the R-loops at centromeres may present RPA-coated ssDNA for ATR activation. This ensures that ATR is specifically activated in prometaphase at centromeres (Kabeche et al., 2018). Together, these data revealed that RPA-mediated recruitment of RNaseH1 and ATR eliminates the aberrant accumulation of R-loops to suppress genomic instability in the S phase, while RPA bound on R-loops at centromeres is important to activate ATR in prometaphase to ensure faithful chromosome segregation (Kabeche et al., 2018; Matos et al., 2020; Nguyen et al., 2018).
RPA interplays with the ubiquitin ligase RFWD3 at stalled forks or DNA lesion sites
RPA is post-translationally modified by phosphorylation, acetylation, sumoylation, or ubiquitination in response to DNA damage or upon fork stalling (Cheng et al., 2018; Dou et al., 2010; Wu et al., 2005). Elia et al. reported that RPA is ubiquitinated by the E3 ligase RFWD3 in response to UV, camptothecin, hydroxyurea and aphidicolin, but not IR treatment, suggesting that fork stalling promotes RPA ubiquitination (Elia et al., 2015). Depletion of RFWD3 impairs fork restart and γH2AX resolution. Using the Tr-GFP reporter, which causes site-specific bidirectional replication fork arrest, the authors demonstrated that depletion of RFWD3 compromises HR repair after Tus-induced fork stalling or I-SceI-induced DSBs (Elia et al., 2015). Furthermore, specific disruption of the interaction between RPA2 and RFWD3 causes inhibition of HU-induced RPA ubiquitination followed by delayed γH2AX resolution and impaired HR at stalled forks. Interestingly, either depletion of RFWD3 or specific disruption of the RPA–RFWD3 interaction causes defective ATR-mediated RPA2 phosphorylation, suggesting the dependence of RPA phosphorylation on its ubiquitination (Elia et al., 2015; Liu et al., 2011).
An independent study by Inano et al. provided evidence that the ATR/ATM-mediated activation of RFWD3 regulates RPA turnover, which ensures the timely removal of RPA from DNA damage sites to promote HR repair (Inano et al., 2017). Inactivation of RFWD3 results in persistent RPA and RAD51 foci after mitomycin (MMC) or IR treatment (Inano et al., 2017). However, the activation of RFWD3 appears to require the phosphorylation of RFWD3 on Serine 46/63 by ATR/ATM, since overexpressing the non-phosphorylable RFWD3–S46/63A mutant compromises RPA ubiquitination (Inano et al., 2017). VCP, a homohexameric molecular chaperone that escorts ubiquitinated proteins to the 26S proteasome for degradation, was found to interact with RPA in response to MMC treatment in an RFWD3-dependent manner. The author further revealed that knockdown of RFWD3, depletion of VCP or expression of the ubiquitination-deficient RPA2-5KR mutant drastically reduces protein turnover of RPA (Inano et al., 2017). Intriguingly, RFWD3 also ubiquitinates RAD51 after MMC damage, leading to its degradation. Finally, the authors concluded that the ubiquitination and turnover of RPA and RAD51 at damage sites are important to repair the DNA damage by HR (Inano et al., 2017). RFWD3-mediated RPA ubiquitination and turnover appear to be also required for proper DNA replication (Lin et al., 2018). Similarly, Feeney et al. reported that RPA-mediated recruitment of RFWD3 to DNA lesion sites plays an important role in the repair of DNA interstrand crosslinks (Feeney et al., 2017). The RFWD3-I639K mutation, which disrupts the interaction between RFWD3 and RPA, impairs RFWD3 recruitment and the repair of interstrand crosslinks and is associated with Fanconi anemia syndrome (Feeney et al., 2017). Thus, RFWD3-mediated RPA turnover is important for the repair of a wide range of DNA lesions.
Concluding remarks
As the sensor of ssDNA, RPA is placed in the center for mediating or coordinating different DNA transactions (Caldwell & Spies, 2020). Recent studies have revealed several important new functions of RPA, extending our understanding of how this complex acts to preserve genome integrity. Interestingly, although RPA has a high affinity for ssDNA, its dynamic association with ssDNA or chromatin requires additional regulations (Li et al., 2018; Peng et al., 2021; Wang et al., 2021). However, how the dynamic binding of RPA on ssDNA or proteins is regulated to ensure proper transferring of a DNA intermediate to the correct repair pathway remains to be elucidated. The emergence of high-resolution single-molecule or structural techniques should allow studying its regulation in detail. RPA is known to interact with a large number of proteins, as documented in two excellent reviews (Caldwell & Spies, 2020; Marechal & Zou, 2015). An important future task is to fully characterize the physiological function and regulation of these interactions, and to address how these interactions may affect the ssDNA-binding mode of RPA or its roles in different DNA metabolic processes.
RPA is tightly associated with carcinogenesis. RPA mutations or improper binding of RPA on ssDNA can impede normal DNA metabolism and cause high levels of spontaneous mutations and genome instability that drive cancer development (Chen & Wold, 2014; Chen et al., 1998; Marechal & Zou, 2015; Martincorena & Campbell, 2015; Tishkoff et al., 1997; Toledo et al., 2013; Wold, 1997). On the other hand, inhibition of RPA or its downstream pathways also impairs the drug sensitivity of several cancer cells (Belanger et al., 2018; Pedersen et al., 2020). Thus, understanding the functions and mechanisms of RPA in different DNA metabolic processes could create new therapeutic strategies for targeting cancers or other diseases.
References
Acharya, A., Kasaciunaite, K., Gose, M., Kissling, V., Guerois, R., Seidel, R., & Cejka, P. (2021). Distinct RPA domains promote recruitment and the helicase-nuclease activities of Dna2. Nature Communications, 12, 6521.
Alberts, B. M., & Frey, L. (1970). T4 bacteriophage gene 32: A structural protein in the replication and recombination of DNA. Nature, 227, 1313–1318.
Alexeev, A., Mazin, A., & Kowalczykowski, S. C. (2003). Rad54 protein possesses chromatin-remodeling activity stimulated by the Rad51-ssDNA nucleoprotein filament. Natural Structural Biology, 10, 182–186.
Amitani, I., Baskin, R. J., & Kowalczykowski, S. C. (2006). Visualization of Rad54, a chromatin remodeling protein, translocating on single DNA molecules. Molecular Cell, 23, 143–148.
Arunkumar, A. I., Stauffer, M. E., Bochkareva, E., Bochkarev, A., & Chazin, W. J. (2003). Independent and coordinated functions of replication protein A tandem high affinity single-stranded DNA binding domains. Journal of Biological Chemistry, 278, 41077–41082.
Bae, S. H., Bae, K. H., Kim, J. A., & Seo, Y. S. (2001). RPA governs endonuclease switching during processing of Okazaki fragments in eukaryotes. Nature, 412, 456–461.
Belanger, F., Fortier, E., Dube, M., Lemay, J. F., Buisson, R., Masson, J. Y., Elsherbiny, A., Costantino, S., Carmona, E., Mes-Masson, A. M., et al. (2018). Replication protein A availability during DNA replication stress is a major determinant of cisplatin resistance in ovarian cancer cells. Cancer Research, 78, 5561–5573.
Bhat, K. P., & Cortez, D. (2018). RPA and RAD51: Fork reversal, fork protection, and genome stability. Nature Structural and Molecular Biology, 25, 446–453.
Brill, S. J., & Stillman, B. (1989). Yeast replication factor-A functions in the unwinding of the SV40 origin of DNA replication. Nature, 342, 92–95.
Brill, S. J., & Stillman, B. (1991). Replication factor-A from Saccharomyces cerevisiae is encoded by three essential genes coordinately expressed at S phase. Genes and Development, 5, 1589–1600.
Byrne, B. M., & Oakley, G. G. (2019). Replication protein A, the laxative that keeps DNA regular: The importance of RPA phosphorylation in maintaining genome stability. Seminars in Cell and Developmental Biology, 86, 112–120.
Caldwell, C. C., & Spies, M. (2020). Dynamic elements of replication protein A at the crossroads of DNA replication, recombination, and repair. Critical Reviews in Biochemistry and Molecular Biology, 55, 482–507.
Ceballos, S. J., & Heyer, W. D. (2011). Functions of the Snf2/Swi2 family Rad54 motor protein in homologous recombination. Biochimica Et Biophysica Acta, 1809, 509–523.
Cejka, P., Cannavo, E., Polaczek, P., Masuda-Sasa, T., Pokharel, S., Campbell, J. L., & Kowalczykowski, S. C. (2010). DNA end resection by Dna2-Sgs1-RPA and its stimulation by Top3-Rmi1 and Mre11-Rad50-Xrs2. Nature, 467, 112–116.
Chen, C., Umezu, K., & Kolodner, R. D. (1998). Chromosomal rearrangements occur in S. cerevisiae rfa1 mutator mutants due to mutagenic lesions processed by double-strand-break repair. Molecular Cell, 2, 9–22.
Chen, H., Lisby, M., & Symington, L. S. (2013). RPA coordinates DNA end resection and prevents formation of DNA hairpins. Molecular Cell, 50, 589–600.
Chen, R., & Wold, M. S. (2014). Replication protein A: Single-stranded DNA’s first responder: Dynamic DNA-interactions allow replication protein A to direct single-strand DNA intermediates into different pathways for synthesis or repair. BioEssays : News and Reviews in Molecular, Cellular and Developmental Biology, 36, 1156–1161.
Cheng, X., Jobin-Robitaille, O., Billon, P., Buisson, R., Niu, H., Lacoste, N., Abshiru, N., Côté, V., Thibault, P., Kron, S. J., et al. (2018). Phospho-dependent recruitment of the yeast NuA4 acetyltransferase complex by MRX at DNA breaks regulates RPA dynamics during resection. Proc Natl Acad Sci U S A, 115, 10028–10033.
Chernikova, S. B., Dorth, J. A., Razorenova, O. V., Game, J. C., & Brown, J. M. (2010). Deficiency in Bre1 impairs homologous recombination repair and cell cycle checkpoint response to radiation damage in mammalian cells. Radiation Research, 174, 558–565.
Chernikova, S. B., Razorenova, O. V., Higgins, J. P., Sishc, B. J., Nicolau, M., Dorth, J. A., Chernikova, D. A., Kwok, S., Brooks, J. D., Bailey, S. M., et al. (2012). Deficiency in mammalian histone H2B ubiquitin ligase Bre1 (Rnf20/Rnf40) leads to replication stress and chromosomal instability. Cancer Research, 72, 2111–2119.
Crickard, J. B., Moevus, C. J., Kwon, Y., Sung, P., & Greene, E. C. (2020a). Rad54 drives ATP hydrolysis-dependent DNA sequence alignment during homologous recombination. Cell, 181, 1380-1394.e1318.
Crossley, M. P., Bocek, M., & Cimprich, K. A. (2019). R-loops as cellular regulators and genomic threats. Molecular Cell, 73, 398–411.
de Vries, J., & Wackernagel, W. (1993). Cloning and sequencing of the Serratia marcescens gene encoding a single-stranded DNA-binding protein (SSB) and its promoter region. Gene, 127, 39–45.
Deng, S. K., Gibb, B., de Almeida, M. J., Greene, E. C., & Symington, L. S. (2014). RPA antagonizes microhomology-mediated repair of DNA double-strand breaks. Nature Structural and Molecular Biology, 21, 405–412.
Dornreiter, I., Erdile, L. F., Gilbert, I. U., von Winkler, D., Kelly, T. J., & Fanning, E. (1992). Interaction of DNA polymerase alpha-primase with cellular replication protein A and SV40 T antigen. EMBO Journal, 11, 769–776.
Dou, H., Huang, C., Singh, M., Carpenter, P. B., & Yeh, E. T. (2010). Regulation of DNA repair through deSUMOylation and SUMOylation of replication protein A complex. Molecular Cell, 39, 333–345.
Elia, A. E., Wang, D. C., Willis, N. A., Boardman, A. P., Hajdu, I., Adeyemi, R. O., Lowry, E., Gygi, S. P., Scully, R., & Elledge, S. J. (2015). RFWD3-dependent ubiquitination of RPA regulates repair at stalled replication forks. Molecular Cell, 60, 280–293.
Fairman, M. P., & Stillman, B. (1988). Cellular factors required for multiple stages of SV40 DNA replication in vitro. EMBO Journal, 7, 1211–1218.
Fan, J., & Pavletich, N. P. (2012). Structure and conformational change of a replication protein A heterotrimer bound to ssDNA. Genes and Development, 26, 2337–2347.
Fanning, E., Klimovich, V., & Nager, A. R. (2006). A dynamic model for replication protein A (RPA) function in DNA processing pathways. Nucleic Acids Research, 34, 4126–4137.
Fay, P. J., Johanson, K., McHenry, C., & Bambara, R. A. (1982). Size classes of products synthesized processively by two subassemblies of Escherichia coli DNA polymerase III holoenzyme. Journal of Biological Chemistry, 257, 5692–5699.
Feeney, L., Munoz, I. M., Lachaud, C., Toth, R., Appleton, P. L., Schindler, D., & Rouse, J. (2017). RPA-mediated recruitment of the E3 Ligase RFWD3 is vital for interstrand crosslink repair and human health. Molecular Cell, 66, 610-621.e614.
Feng, S., & Manley, J. L. (2021). Replication protein A associates with nucleolar R loops and regulates rRNA transcription and nucleolar morphology. Genes & Development, 35, 1579–1594.
Fierz, B., Chatterjee, C., McGinty, R. K., Bar-Dagan, M., Raleigh, D. P., & Muir, T. W. (2011). Histone H2B ubiquitylation disrupts local and higher-order chromatin compaction. Nature Chemical Biology, 7, 113–119.
Fuchs, G., & Oren, M. (2014). Writing and reading H2B monoubiquitylation. Biochimica Et Biophysica Acta, 1839, 694–701.
Garcia-Muse, T., & Aguilera, A. (2019). R Loops: From Physiological to Pathological Roles. Cell, 179, 604–618.
Gibb, B., Ye, L. F., Kwon, Y., Niu, H., Sung, P., & Greene, E. C. (2014). Protein dynamics during presynaptic-complex assembly on individual single-stranded DNA molecules. Nature Structural & Molecular Biology, 21, 893–900.
Gorman, J., Chowdhury, A., Surtees, J. A., Shimada, J., Reichman, D. R., Alani, E., & Greene, E. C. (2007). Dynamic basis for one-dimensional DNA scanning by the mismatch repair complex Msh2-Msh6. Molecular Cell, 28, 359–370.
Greene, E. C., Wind, S., Fazio, T., Gorman, J., & Visnapuu, M. L. (2010). DNA curtains for high-throughput single-molecule optical imaging. Methods in Enzymology, 472, 293–315.
Grummt, I. (2013). The nucleolus-guardian of cellular homeostasis and genome integrity. Chromosoma, 122, 487–497.
Gurard-Levin, Z. A., Quivy, J.-P., & Almouzni, G. (2014). Histone chaperones: Assisting histone traffic and nucleosome dynamics. Annual Review of Biochemistry, 83, 487–517.
Haber, J. E. (2016). A Life Investigating Pathways That Repair Broken Chromosomes. Annual Review of Genetics, 50, 1–28.
Hass, C. S., Gakhar, L., & Wold, M. S. (2010). Functional characterization of a cancer causing mutation in human replication protein A. Molecular Cancer Research, 8, 1017–1026.
Hegazy, Y. A., Fernando, C. M., & Tran, E. J. (2020). The balancing act of R-loop biology: The good, the bad, and the ugly. Journal of Biological Chemistry, 295, 905–913.
Huang, S., Zhou, H., Katzmann, D., Hochstrasser, M., Atanasova, E., & Zhang, Z. (2005). Rtt106p is a histone chaperone involved in heterochromatin-mediated silencing. Proc Natl Acad Sci U S A, 102, 13410–13415.
Hung, S. H., Wong, R. P., Ulrich, H. D., & Kao, C. F. (2017). Monoubiquitylation of histone H2B contributes to the bypass of DNA damage during and after DNA replication. Proc Natl Acad Sci U S A, 114, E2205–E2214.
Hwang, W. W., Venkatasubrahmanyam, S., Ianculescu, A. G., Tong, A., Boone, C., & Madhani, H. D. (2003). A conserved RING finger protein required for histone H2B monoubiquitination and cell size control. Molecular Cell, 11, 261–266.
Iftode, C., Daniely, Y., & Borowiec, J. A. (1999). Replication protein A (RPA): The eukaryotic SSB. Critical Reviews in Biochemistry and Molecular Biology, 34, 141–180.
Inano, S., Sato, K., Katsuki, Y., Kobayashi, W., Tanaka, H., Nakajima, K., Nakada, S., Miyoshi, H., Knies, K., Takaori-Kondo, A., et al. (2017). RFWD3-mediated ubiquitination promotes timely removal of both RPA and RAD51 from DNA damage sites to facilitate homologous recombination. Molecular Cell, 66, 622-634.e628.
Kabeche, L., Nguyen, H. D., Buisson, R., & Zou, L. (2018). A mitosis-specific and R loop-driven ATR pathway promotes faithful chromosome segregation. Science, 359, 108–114.
Kowalczykowski, S. C. (1991). Biochemical and biological function of Escherichia coli RecA protein: Behavior of mutant RecA proteins. Biochimie, 73, 289–304.
Kowalczykowski, S. C. (2015). An Overview of the Molecular Mechanisms of Recombinational DNA Repair. Cold Spring Harbor Perspectives in Biology, 7, a016410.
Krasikova, Y. S., Rechkunova, N. I., & Lavrik, O. I. (2016). Replication protein A as a major eukaryotic single-stranded DNA-binding protein and its role in DNA repair. Molekuliarnaia Biologiia (mosk), 50, 735–750.
Li, S., Xu, Z., Xu, J., Zuo, L., Yu, C., Zheng, P., Gan, H., Wang, X., Li, L., Sharma, S., et al. (2018). Rtt105 functions as a chaperone for replication protein A to preserve genome stability. EMBO J 37.
Li, G. M. (2008). Mechanisms and functions of DNA mismatch repair. Cell Research, 18, 85–98.
Lin, Y. C., Wang, Y., Hsu, R., Giri, S., Wopat, S., Arif, M. K., Chakraborty, A., Prasanth, K. V., & Prasanth, S. G. (2018). PCNA-mediated stabilization of E3 ligase RFWD3 at the replication fork is essential for DNA replication. Proc Natl Acad Sci U S A, 115, 13282–13287.
Liu, G., Yan, J., Wang, X., Chen, J., Wang, X., Dong, Y., Zhang, S., Gan, X., Huang, J., and Chen, X. (2021a). RPA-mediated recruitment of Bre1 couples histone H2B ubiquitination to DNA replication and repair. Proc Natl Acad Sci U S A 118.
Liu, S., Chu, J., Yucer, N., Leng, M., Wang, S. Y., Chen, B. P., Hittelman, W. N., & Wang, Y. (2011). RING finger and WD repeat domain 3 (RFWD3) associates with replication protein A (RPA) and facilitates RPA-mediated DNA damage response. Journal of Biological Chemistry, 286, 22314–22322.
Liu, S., Hua, Y., Wang, J., Li, L., Yuan, J., Zhang, B., Wang, Z., Ji, J., & Kong, D. (2021b). RNA polymerase III is required for the repair of DNA double-strand breaks by homologous recombination. Cell, 184, 1314-1329.e1310.
Liu, S., & Kong, D. (2021). A direct role of RNA polymerase III and RNA in DNA homologous recombination. Mol Cell Oncol, 8, 1935173.
Liu, S., Xu, Z., Leng, H., Zheng, P., Yang, J., Chen, K., Feng, J., & Li, Q. (2017). RPA binds histone H3–H4 and functions in DNA replication-coupled nucleosome assembly. Science, 355, 415–420.
Lohman, T. M., & Ferrari, M. E. (1994). Escherichia coli single-stranded DNA-binding protein: Multiple DNA-binding modes and cooperativities. Annual Review of Biochemistry, 63, 527–570.
Marechal, A., & Zou, L. (2015). RPA-coated single-stranded DNA as a platform for post-translational modifications in the DNA damage response. Cell Research, 25, 9–23.
Marnef, A., Cohen, S., & Legube, G. (2017). Transcription-coupled DNA double-strand break repair: Active genes need special care. Journal of Molecular Biology, 429, 1277–1288.
Martincorena, I., & Campbell, P. J. (2015). Somatic mutation in cancer and normal cells. Science, 349, 1483–1489.
Matos, D. A., Zhang, J. M., Ouyang, J., Nguyen, H. D., Genois, M. M., & Zou, L. (2020). ATR protects the genome against R loops through a MUS81-triggered feedback loop. Molecular Cell, 77, 514-527.e514.
McVey, M., & Lee, S. E. (2008). MMEJ repair of double-strand breaks (director’s cut): Deleted sequences and alternative endings. Trends in Genetics, 24, 529–538.
Mehta, A., Beach, A., & Haber, J. E. (2017). Homology requirements and competition between gene conversion and break-induced replication during double-strand break repair. Molecular Cell, 65, 515-526.e513.
Molineux, I. J., Friedman, S., & Gefter, M. L. (1974). Purification and properties of the Escherichia coli deoxyribonucleic acid-unwinding protein: Effects on deoxyribonucleic acid synthesis in vitro. Journal of Biological Chemistry, 249, 6090–6098.
Moyal, L., Lerenthal, Y., Gana-Weisz, M., Mass, G., So, S., Wang, S. Y., Eppink, B., Chung, Y. M., Shalev, G., Shema, E., et al. (2011). Requirement of ATM-dependent monoubiquitylation of histone H2B for timely repair of DNA double-strand breaks. Molecular Cell, 41, 529–542.
Nakamura, K., Kato, A., Kobayashi, J., Yanagihara, H., Sakamoto, S., Oliveira, D. V., Shimada, M., Tauchi, H., Suzuki, H., Tashiro, S., et al. (2011). Regulation of homologous recombination by RNF20-dependent H2B ubiquitination. Molecular Cell, 41, 515–528.
Nguyen, H. D., Leong, W. Y., Li, W., Reddy, P. N. G., Sullivan, J. D., Walter, M. J., Zou, L., & Graubert, T. A. (2018). Spliceosome mutations induce R loop-associated sensitivity to ATR inhibition in myelodysplastic syndromes. Cancer Research, 78, 5363–5374.
Nguyen, H. D., Yadav, T., Giri, S., Saez, B., Graubert, T. A., & Zou, L. (2017). Functions of replication protein A as a sensor of r loops and a regulator of RNaseH1. Molecular Cell, 65, 832-847.e834.
Niehrs, C., & Luke, B. (2020). Regulatory R-loops as facilitators of gene expression and genome stability. Nature Rev Mol Cell Biol, 21, 167–178.
Nimonkar, A. V., Genschel, J., Kinoshita, E., Polaczek, P., Campbell, J. L., Wyman, C., Modrich, P., & Kowalczykowski, S. C. (2011). BLM-DNA2-RPA-MRN and EXO1-BLM-RPA-MRN constitute two DNA end resection machineries for human DNA break repair. Genes and Development, 25, 350–362.
Niu, H., Chung, W. H., Zhu, Z., Kwon, Y., Zhao, W., Chi, P., Prakash, R., Seong, C., Liu, D., Lu, L., et al. (2010). Mechanism of the ATP-dependent DNA end-resection machinery from Saccharomyces cerevisiae. Nature, 467, 108–111.
Northam, M. R., & Trujillo, K. M. (2016). Histone H2B mono-ubiquitylation maintains genomic integrity at stalled replication forks. Nucleic Acids Research, 44, 9245–9255.
Pedersen, H., Anne Adanma Obara, E., Elbæk, K. J., Vitting-Serup, K., & Hamerlik, P. (2020). Replication protein A (RPA) mediates radio-resistance of glioblastoma cancer stem-like cells. International Journal of Molecular Sciences, 21, 1588.
Peng, H., Zhang, S., Peng, Y., Zhu, S., Zhao, X., Zhao, X., Yang, S., Liu, G., Dong, Y., Gan, X., et al. (2021). Yeast bromodomain factor 1 and its human homolog TAF1 play conserved roles in promoting homologous recombination. Adv Sci (weinh), 8, e2100753.
Petukhova, G., Stratton, S., & Sung, P. (1998). Catalysis of homologous DNA pairing by yeast Rad51 and Rad54 proteins. Nature, 393, 91–94.
Pokhrel, N., Caldwell, C. C., Corless, E. I., Tillison, E. A., Tibbs, J., Jocic, N., Tabei, S. M. A., Wold, M. S., Spies, M., & Antony, E. (2019). Dynamics and selective remodeling of the DNA-binding domains of RPA. Nature Structural and Molecular Biology, 26, 129–136.
Prasad, T. K., Robertson, R. B., Visnapuu, M. L., Chi, P., Sung, P., & Greene, E. C. (2007). A DNA-translocating Snf2 molecular motor: Saccharomyces cerevisiae Rdh54 displays processive translocation and extrudes DNA loops. Journal of Molecular Biology, 369, 940–953.
Ristic, D., Wyman, C., Paulusma, C., & Kanaar, R. (2001). The architecture of the human Rad54-DNA complex provides evidence for protein translocation along DNA. Proc Natl Acad Sci U S A, 98, 8454–8460.
Robzyk, K., Recht, J., & Osley, M. A. (2000). Rad6-dependent ubiquitination of histone H2B in yeast. Science, 287, 501–504.
Roca, A. I., & Cox, M. M. (1990). The RecA protein: Structure and function. Critical Reviews in Biochemistry and Molecular Biology, 25, 415–456.
Ruff, P., Donnianni, R. A., Glancy, E., Oh, J., & Symington, L. S. (2016). RPA stabilization of single-stranded DNA is critical for break-induced replication. Cell Reports, 17, 3359–3368.
Schramke, V., Luciano, P., Brevet, V., Guillot, S., Corda, Y., Longhese, M. P., Gilson, E., & Geli, V. (2004). RPA regulates telomerase action by providing Est1p access to chromosome ends. Nature Genetics, 36, 46–54.
Shen, J., Zhao, Y., Pham, N. T., Li, Y., Zhang, Y., Trinidad, J., Ira, G., Qi, Z., & Niu, H. (2022). Deciphering the mechanism of processive ssDNA digestion by the Dna2-RPA ensemble. Nature Communications, 13, 359.
Sigal, N., Delius, H., Kornberg, T., Gefter, M. L., & Alberts, B. (1972). A DNA-unwinding protein isolated from Escherichia coli: Its interaction with DNA and with DNA polymerases. Proc Natl Acad Sci U S A, 69, 3537–3541.
Smith, S., & Stillman, B. (1989). Purification and characterization of CAF-I, a human cell factor required for chromatin assembly during DNA replication in vitro. Cell, 58, 15–25.
Sugiyama, T., New, J. H., & Kowalczykowski, S. C. (1998). DNA annealing by RAD52 protein is stimulated by specific interaction with the complex of replication protein A and single-stranded DNA. Proc Natl Acad Sci U S A, 95, 6049–6054.
Sun, Z. W., & Allis, C. D. (2002). Ubiquitination of histone H2B regulates H3 methylation and gene silencing in yeast. Nature, 418, 104–108.
Symington, L. S., Rothstein, R., & Lisby, M. (2014). Mechanisms and regulation of mitotic recombination in Saccharomyces cerevisiae. Genetics, 198, 795–835.
Tishkoff, D. X., Filosi, N., Gaida, G. M., & Kolodner, R. D. (1997). A novel mutation avoidance mechanism dependent on S. cerevisiae RAD27 is distinct from DNA mismatch repair. Cell, 88, 253–263.
Toledo, L. I., Altmeyer, M., Rask, M. B., Lukas, C., Larsen, D. H., Povlsen, L. K., Bekker-Jensen, S., Mailand, N., Bartek, J., & Lukas, J. (2013). ATR prohibits replication catastrophe by preventing global exhaustion of RPA. Cell, 155, 1088–1103.
Trujillo, K. M., & Osley, M. A. (2012). A role for H2B ubiquitylation in DNA replication. Molecular Cell, 48, 734–746.
Tyler, J. K., Adams, C. R., Chen, S. R., Kobayashi, R., Kamakaka, R. T., & Kadonaga, J. T. (1999). The RCAF complex mediates chromatin assembly during DNA replication and repair. Nature, 402, 555–560.
VanDemark, A. P., Blanksma, M., Ferris, E., Heroux, A., Hill, C. P., & Formosa, T. (2006). The structure of the yFACT Pob3-M domain, its interaction with the DNA replication factor RPA, and a potential role in nucleosome deposition. Molecular Cell, 22, 363–374.
Visnapuu, M. L., & Greene, E. C. (2009). Single-molecule imaging of DNA curtains reveals intrinsic energy landscapes for nucleosome deposition. Nature Structural and Molecular Biology, 16, 1056–1062.
Waga, S., & Stillman, B. (1994). Anatomy of a DNA replication fork revealed by reconstitution of SV40 DNA replication in vitro. Nature, 369, 207–212.
Wang, X., Dong, Y., Zhao, X., Li, J., Lee, J., Yan, Z., Yang, S., Wu, W., Hou, X., Liu, G., et al. (2021). Rtt105 promotes high-fidelity DNA replication and repair by regulating the single-stranded DNA-binding factor RPA. Proc Natl Acad Sci U S A, 118, e2106393118.
Wobbe, C. R., Weissbach, L., Borowiec, J. A., Dean, F. B., Murakami, Y., Bullock, P., & Hurwitz, J. (1987). Replication of simian virus 40 origin-containing DNA in vitro with purified proteins. Proc Natl Acad Sci U S A, 84, 1834–1838.
Wold, M. S. (1997). Replication protein A: A heterotrimeric, single-stranded DNA-binding protein required for eukaryotic DNA metabolism. Annual Review of Biochemistry, 66, 61–92.
Wold, M. S., & Kelly, T. (1988). Purification and characterization of replication protein A, a cellular protein required for in vitro replication of simian virus 40 DNA. Proc Natl Acad Sci U S A, 85, 2523–2527.
Wood, A., Krogan, N. J., Dover, J., Schneider, J., Heidt, J., Boateng, M. A., Dean, K., Golshani, A., Zhang, Y., Greenblatt, J. F., et al. (2003). Bre1, an E3 ubiquitin ligase required for recruitment and substrate selection of Rad6 at a promoter. Molecular Cell, 11, 267–274.
Wright, W. D., & Heyer, W. D. (2014). Rad54 functions as a heteroduplex DNA pump modulated by its DNA substrates and Rad51 during D loop formation. Molecular Cell, 53, 420–432.
Wu, X., Yang, Z., Liu, Y., & Zou, Y. (2005). Preferential localization of hyperphosphorylated replication protein A to double-strand break repair and checkpoint complexes upon DNA damage. The Biochemical Journal, 391, 473–480.
Xu, Z., Feng, J., & Li, Q. (2021). Measuring genome-wide nascent nucleosome assembly using ReIN-Map. In Yeast Protocols (pp. 117–141). Springer.
Yang, J., Zhang, X., Feng, J., Leng, H., Li, S., Xiao, J., Liu, S., Xu, Z., Xu, J., Li, D., et al. (2016). The histone chaperone FACT contributes to DNA replication-coupled nucleosome assembly. Cell Reports, 16, 3414.
Yuzhakov, A., Kelman, Z., Hurwitz, J., & O’Donnell, M. (1999). Multiple competition reactions for RPA order the assembly of the DNA polymerase delta holoenzyme. The EMBO Journal, 18, 6189–6199.
Zeng, M., Ren, L., Mizuno, K., Nestoras, K., Wang, H., Tang, Z., Guo, L., Kong, D., Hu, Q., He, Q., et al. (2016). CRL4(Wdr70) regulates H2B monoubiquitination and facilitates Exo1-dependent resection. Nature Communications, 7, 11364.
Zhang, Z., Fan, H. Y., Goldman, J. A., & Kingston, R. E. (2007). Homology-driven chromatin remodeling by human RAD54. Nature Structural and Molecular Biology, 14, 397–405.
Zheng, L., Meng, Y., Campbell, J. L., & Shen, B. (2020). Multiple roles of DNA2 nuclease/helicase in DNA metabolism, genome stability and human diseases. Nucleic Acids Research, 48, 16–35.
Zheng, S., Li, D., Lu, Z., Liu, G., Wang, M., Xing, P., Wang, M., Dong, Y., Wang, X., Li, J., et al. (2018). Bre1-dependent H2B ubiquitination promotes homologous recombination by stimulating histone eviction at DNA breaks. Nucleic Acids Research, 46, 11326–11339.
Zhu, B., Zheng, Y., Pham, A. D., Mandal, S. S., Erdjument-Bromage, H., Tempst, P., & Reinberg, D. (2005). Monoubiquitination of human histone H2B: The factors involved and their roles in HOX gene regulation. Molecular Cell, 20, 601–611.
Zhu, Z., Chung, W. H., Shim, E. Y., Lee, S. E., & Ira, G. (2008). Sgs1 helicase and two nucleases Dna2 and Exo1 resect DNA double-strand break ends. Cell, 134, 981–994.
Zou, L., & Elledge, S. J. (2003). Sensing DNA damage through ATRIP recognition of RPA-ssDNA complexes. Science, 300, 1542–1548.
Zou, Y., Liu, Y., Wu, X., & Shell, S. M. (2006). Functions of human replication protein A (RPA): From DNA replication to DNA damage and stress responses. Journal of Cellular Physiology, 208, 267–273.
Acknowledgements
This research was supported by grants from the National Natural Science Foundation of China [32070573, 31872808 and 31671294], the National Key Research and Development Program of China [2021YFA1100503], TaiKang Center for Life and Medical Sciences and Wuhan University Advanced Genetics Course Program to X.C.
Author information
Authors and Affiliations
Hubei Key Laboratory of Cell Homeostasis, College of Life Sciences, TaiKang Center for Life and Medical Sciences, Frontier Science Centre of Immunology and Metabolism, Wuhan University, Wuhan, 430072, China
Simin Zhang, Xuejie Wang, Han Zhao, Jingyao Shi & Xuefeng Chen
Corresponding author
Correspondence to Xuefeng Chen.
Ethics declarations
Conflict of interest
The authors declare no competing interests.
Rights and permissions
Springer Nature or its licensor holds exclusive rights to this article under a publishing agreement with the author(s) or other rightsholder(s); author self-archiving of the accepted manuscript version of this article is solely governed by the terms of such publishing agreement and applicable law.
About this article
Cite this article
Zhang, S., Wang, X., Zhao, H. et al. New insights into the mechanism of RPA in preserving genome stability. GENOME INSTAB. DIS. 3, 255–266 (2022). https://doi.org/10.1007/s42764-022-00085-y
Received31 July 2022
Revised18 September 2022
Accepted20 September 2022
Published29 September 2022
Issue DateOctober 2022
DOIhttps://doi.org/10.1007/s42764-022-00085-y
Share this article
Anyone you share the following link with will be able to read this content:
Get shareable linkKeywords
RPA
ssDNA
Genome stability
DNA replication
DNA repair
用户登录
还没有账号?
立即注册