Mitochondrial DNA mutation affects the pluripotency of embryonic stem cells with metabolism modulation
Original Research Paper
Juntao Qi, Qi Long, Yang Yuan, Yanshuang Zhou, Jian Zhang, Zifeng Ruan, Liang Yang, Yi Wu, Ge Xiang, Wei Li, Hao Wu, Shiwei Du & Xingguo Liu
Genome Instability & Disease (2022)
Abstract
Mitochondria are the only organelles other than the nucleus harboring their DNA in mammalian cells. The mitochondrial DNA (mtDNA) mutation is the cause of many serious diseases, which still lack effective therapies. Our previous report showed that mtDNA mutation exacerbates female reproductive aging. However, the regulation of mtDNA mutation on stem cells is still unknown. Herein, we generated embryonic stem cells (ESCs) harboring massive mtDNA mutations without affecting genomic DNA to study the role of mtDNA mutation in pluripotency. The mtDNA mutation impacted the balance of pluripotency and totipotency of ESCs with a metabolism modulation as the down-regulation of oxidative phosphorylation in energy production, followed by a high level of ROS production by mitochondria. This work would shed light on the investigation and treatment of mtDNA mutant diseases.
Introduction
Mitochondria are the powerhouse and metabolic center of mammalian cells and the only organelles other than the nucleus contain their own DNA. Mitochondrial DNA (mtDNA) encodes 13 peptides, 22 transfer RNAs (tRNAs), and two ribosomal RNAs (rRNAs), which are critical for mitochondrial metabolism, respiration, transcription, and translation (Anderson et al., 1981; Bayona-Bafaluy et al., 2008; Bibb et al., 1981; van der Bliek et al., 2017). The mtDNA mutations are the main cause of many serious diseases in humans, the prevalence of which is 5–15 cases per 100,000 in childhood (< 16 years old) and 9.6 cases per 100,000 in adults (Russell et al., 2020). These include Leber’s hereditary optic neuropathy (LHON) (Santorelli et al., 1993; Tatuch et al., 1992; Wallace et al., 1988), mitochondrial encephalomyopathy, lactic acidosis, and stroke-like episodes (MELAS) (Goto et al., 1990; Kobayashi et al., 1990), neurodegenerative diseases, and aging (Iyer et al., 2012; Russell et al., 2020).
However, most of these diseases still lack effective treatments due to the lack of disease model (Emperador et al., 2019; Perry et al., 2021; Vafai & Mootha, 2012). In our previous report, we have used a POLG D257A point mutant mouse model to reveal the aging of reproduction induced by mtDNA mutation (Yang et al., 2020). Herein, we generated a mouse embryonic stem cell (mESC) model, harboring mtDNA mutations but no genomic DNA mutations, to study the impacts on the pluripotency of stem cells. The mtDNA mutation impacted the pluripotency as defects in embryoid bodies’ differentiation, while it induced an imbalance between pluripotency and totipotency as metabolism modulation with a down-regulation of oxidative phosphorylation in energy production. Our findings would benefit the study and treatment of mtDNA mutant diseases.
Materials and methods
Animals
PolgAD257A heterozygote mice (PolgAWT/Mut) were purchased from Jackson Laboratory (Stock No. 017341). All animals were handled following the guidelines of the National Institutes of Health for the Care and Use of Laboratory Animals (NIH Publications No. 8023, revised 1978). The study was approved by the Institutional Animal Care and Use Committee of Guangzhou Institutes of Biomedicine and Health, Chinese Academy of Sciences (GIBH). All experiments were performed per the guidelines approved by the Ethical Committee of GIBH.
Cells
PolgAWT/Mut female mice were crossed with wild-type (WT) male mice after receiving 7.5 IU pregnant mare serum gonadotropin (PMSG) (EasyCheck, M2630) and human chorionic gonadotropin (hCG) (EasyCheck, M2530) to obtain mESCs with mtDNA mutations. E3.5 mouse embryos were isolated and seeded in dishes pre-coated with ICR mouse embryo fibroblast (MEF) feeder cells. The cell colonies growing from the inner cell mass were selected and seeded on a new cell culture dish coated with feeder cells. ESC colonies were further cultured and expanded in an mES medium (Al Rawi et al., 2011; Evans & Kaufman, 1981) containing Dulbecco’s modified Eagle’s medium (DMEM)/high glucose (Gibco, 0030034DJ) supplemented with 15% fetal bovine serum (Gibco, 2350404RP), 1% non-essential amino acids (NEAAs) (Gibco, 11140050), 1% GlutaMAX (Gibco, 35050061), 1% sodium pyruvate (Gibco, 11360070), 2-mercaptoethanol (Gibco, 21985023), 1000 U/mL leukemia inhibitory factor (LIF), penicillin/streptomycin (Hyclone, SV30010), CHIR99021 (Selleck, S1263), and PD0325901 (Selleck, S1036). mESCs without feeder cells were cultured on dishes pre-coated with gelatin (StemCell, 07903). The WT mES cell line, R1, was used as the control.
Genotyping
Mouse and ESC genotyping was performed as previously described (Yang et al., 2020). Briefly, whole genomic DNA was extracted using the TIANamp Genomic DNA Kit (Tiangen, B0004DP) and amplified with Polg-D257A-FP and Polg-D257A-RP primers (Table S1). A polymerase chain reaction (PCR) was performed using KOD Plus Polymerase (Toyobo, KOD-201) following the manufacturer’s instructions.
mtDNA sequencing
Whole genomic DNA, including mtDNA, was extracted from ESCs using a TIANamp Genomic DNA Kit (Tiangen, B0004DP). PCR amplification was carried out with Mt1-4 F/R primers (Table S1), and the products were then mixed and sequenced on the Illumina Hiseq4000 platform using 150-bp paired-end reads for 3G flux to sequence the mtDNA. The quality-filtered reads of mtDNA were aligned to the reference mitochondrial genome of the Mus musculus strain C57BL/6J mitochondrion (GenBank: AY172335.1) using default parameters. The sum of the different results was marked as the mutation frequency at a site different from the reference site. A site with a mutation frequency greater than 0.5% was considered as a putative mutation to avoid sporadic sequencing errors (Yang et al., 2020).
Immunofluorescence
Immunofluorescence was performed as previously described (Zhou et al., 2020). Briefly, mESCs seeded on cover glass were fixed with 4% paraformaldehyde (Genesion, JX0100) for 10 min and then permeabilized with 0.5% Triton X-100 (Sigma, T8787) for another 10 min. After blocking with 10% goat serum, the slips were incubated with diluted primary antibodies (Table S2) overnight at 4 °C. Secondary antibodies conjugated to Alexa Fluor 488 or 568 were used and incubated for 2 h at room temperature. The cells were imaged with Zeiss LSM 800 confocal microscopy equipment.
Mitochondrial reactive oxygen species (mtROS)
Cells were incubated with 5 μM MitoSOX Red (Invitrogen, M36008), diluted in Hanks’ Balanced Salt Solution (Beyotime, C0218) for 10 min, and washed twice with Hanks’ solution to detect mtROS. The mESCs cultured without feeder cells were digested into single cells with 0.05% trypsin (Gibco, 25300054), and mtROS were detected by BD LSRFortessa (USA) excitation at 586 nm.
Extracellular metabolic flux analysis
The mitochondrial-stress test was performed following instructions by the manufacturer (XFe24, Seahorse Bioscience). The culture medium was replaced by XF Base Medium (Seahorse Bioscience, 103576-100) supplemented with 1 mM of L-glutamine and 1 mM of pyruvate, 4 h after seeding on the gelatin-coated 24-well plates at a density of 5 × 104 cells/well. Cells were equilibrated for 1 h at 37 °C without a CO2 supply before the test. For the mitochondrial-stress test, the oxygen consumption rate (OCR) was measured step by step after adding 0.5 µM oligomycin, 0.5 µM carbonyl cyanide-p-trifluoromethoxyphenylhydrazone (FCCP) and 0.5 µM rotenone. All reagents were supplied by the kits (Seahorse Bioscience, 103015-100).
Embryoid body (EB) differentiation
ESCs were dissociated with 0.05% Trypsin (Gibco, C25300054) and gently pipetted to disperse the colonies into single cells. The cells were re-suspended to a density of 5.5 × 105 cells/mL and seeded on the cover of the cell culture dish with Knockout DMEM (Gibco, 10829-018) supplemented with 15% Knockout Serum Replacement (Gibco, 10828-028), 1% NEAAs and 1% GlutaMAX after centrifuging for 3 min at 300 g. The cells were cultured for 3 days to generate EBs at 37 °C supplied with 5% CO2 (Evans & Kaufman, 1981; Martin et al., 1981).
Quantitative PCR (qPCR)
Total RNA was extracted using an EZ-press RNA Purification Kit (EZBioscience, B0004D), and 1 μg RNA was used for each sample for reverse transcription. The qPCR reactions were carried out with SYBR Green (Takara, RR430S) and run on a QuantStudio 1 system (ABI). Genomes were diluted to 5 ng/μL, and the mtDNA copy number was calculated using the copy number of Cox2 to Rps18 ratio (Gomes et al., 2013). Table S1 lists all of the primers used.
Data analysis
Data were statistically analyzed using GraphPad PRISM 8.0 and were shown as the mean ± standard deviation (SD). To assess statistical significance, data from three independent experiments were analyzed using one-tailed unpaired Student’s t test (*p < 0.05; **p < 0.01; ***p < 0.001).
Results and discussion
Generating ESCs harboring massive mtDNA mutations
Apart from some patient-derived somatic cells, it is difficult to generate cells harboring mtDNA mutations but without mutations in genomic DNA. Herein, we generated ESCs with substantial mtDNA mutations without affecting genomic DNA in mice (Fig. 1A). The D257A mutation on POLG, the polymerase responsible for mtDNA replication, induces many replication errors, resulting in massive and random mtDNA mutations (Hance et al., 2005; Ropp & Copeland, 1996; Spelbrink et al., 2000). Taking advantage of maternal mitochondrial inheritance, we crossed heterozygous female mice harboring one copy of the mutant Polg gene (PolgAWT/Mut) with wild-type (WT) male mice to harvest E3.5 embryos carrying mtDNA mutations (Kujoth et al., 2005; Trifunovic et al., 2004). The embryos were seeded on feeder cells and ESC colonies were picked out after several days of culturing (Fig. 1B). All the embryos should harbor mutant mitochondria inherited from the maternal mice. Following genotyping, the colonies without Polg mutations were selected and expanded on fresh dishes coated with feeder cells, which were marked as Mitomut ESC (Fig. 1C). The expression of several pluripotency markers, including OCT4, NANOG, REX1 and SSEA1 confirmed in these ES colonies was examined using immunofluorescence assay (Fig. 1D). We further examined ESCs that harbored mtDNA mutations but did not contain genomic DNA mutations.
Fig. 1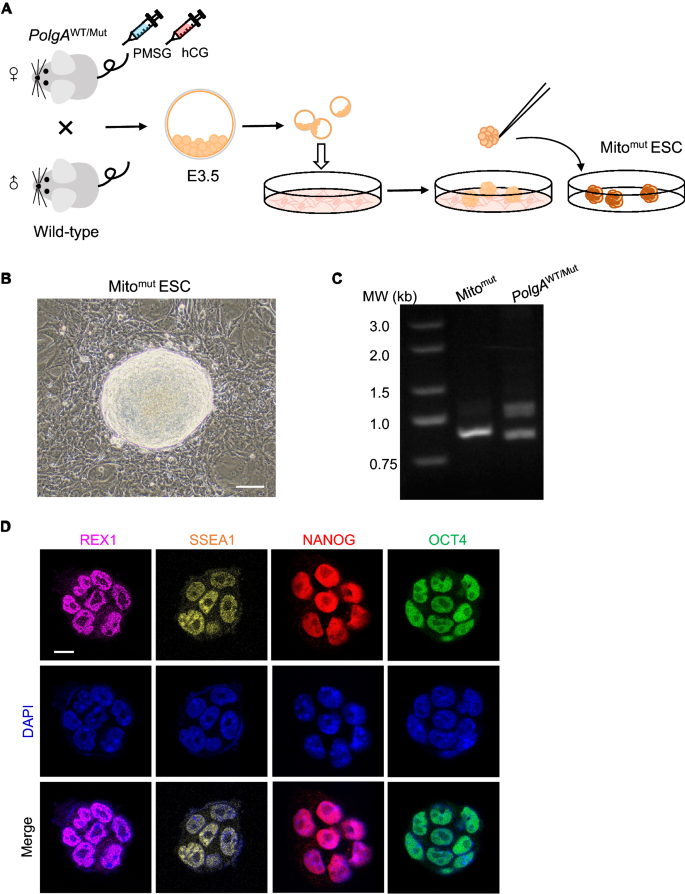
Generating ESCs carrying mtDNA mutations from Polg mutant mice. A Schematic diagram of generating ESCs carrying mtDNA mutations from Polg mutant mice. B Images of the isolated and expanded ESC colonies cultured on feeder cells. Scale bar: 20 μm. C The genotype of the isolated ESC colonies. D Pluripotency markers expression in isolated ESCs detected by the immunofluorescence assay. Scale bar: 10 μm
Identifying mtDNA mutations in the ESC colonies
To pinpoint the mtDNA mutations in these ESC colonies, the mtDNA sequencing was performed in three ESC colonies, including two colonies of Mitomut and one colony with Polg mutation (PolgAWT/Mut) (Fig. 2A). All three colonies shared a lot of substantial mutations as point mutations in mtDNA (Fig. 2B). The mutations were distributed randomly in the 16.3 kb mtDNA, with few peaks in any particular site (Fig. 2A). There were a greater frequency of deletion mutations, and fewer insertion and point mutations were identified in Mitomut-1 (Fig. 2B). Among all types of mutations, single-nucleotide polymorphisms (SNPs) accounted for the majority in prominent as low-frequency random mutations (Fig. 2B, C). We also checked the mutations in 12 peptides and two RNAs encoded by mtDNA, and also identified more mutations on Mitomut-2 than on Mitomut-1 (Fig. 2D, E). Although the Mitomut-2 ESCs colonies showed more similarities to the ESCs with Polg mutations, both two colonies harbored massive mtDNA mutations, but no Polg mutation in genomic DNA.
Fig. 2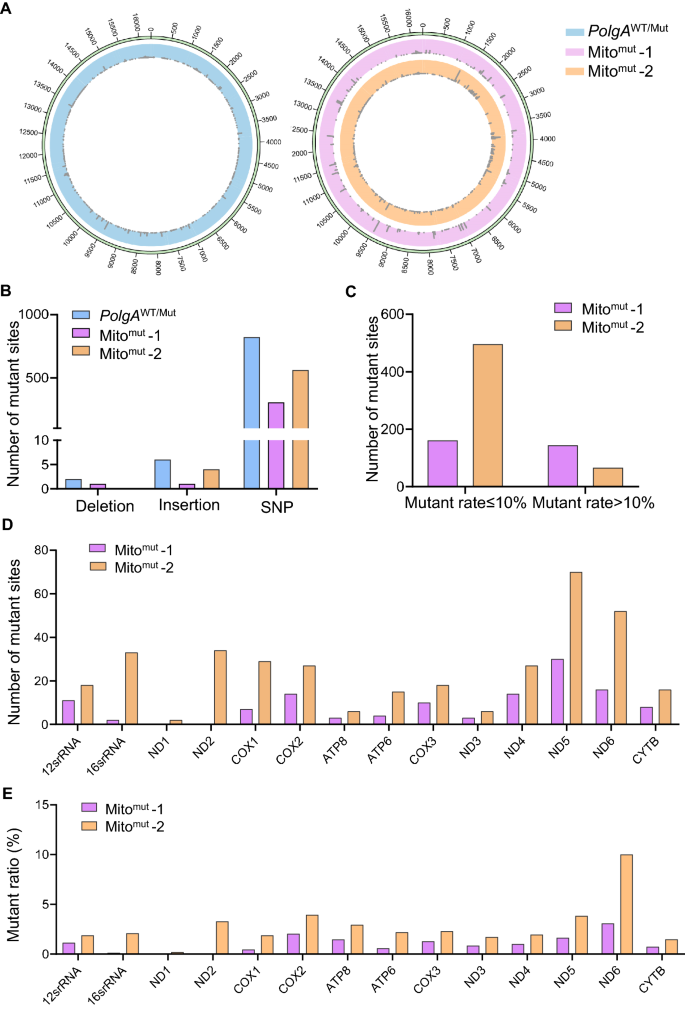
Identifying mtDNA mutations in ESC colonies. A Circular plot showing the mtDNA point mutations in ESC colonies. The reference mtDNA genome is Mus musculus strain C57BL/6J mitochondrion (GenBank: AY172335.1). Mitomut-1 and Mitomut-2 are two ESC colonies with the PolgAWT/WT genotype. The PolgAWT/Mut (left) is set as a positive control. B The number of deletion, insertion, and SNP mutations in the identified ES colonies. C The number of point mutations in the identified ES colonies. The point mutations were divided into high-frequency mutation (mutant rate > 10%) and low-frequency mutation (mutant rate ≤ 10%) groups. D, E The number of mutant sites (D) and the mutant region/the whole gene sequence ratio (E) of the mtDNA coding peptides and RNAs in the identified ESCs
Impacting the pluripotency and metabolism of stem cells by mtDNA mutations
To study the effects of mtDNA mutation on pluripotency of ESCs, we detected the mtDNA copy number and mtDNA expression with qPCR at first. Both Mitomut and WT ESCs have almost the same amount of mtDNA copies, indicating the normal mtDNA replication (Fig. 2A). Meanwhile, a peak up of the mtDNA-coding genes was identified in Mitomut ESCs (Fig. 3B). As the powerhouse of cells, mitochondria with substantial mtDNA mutations would influence the metabolism and growth of stem cells (Ji et al., 2021). Unexpectedly, no significant difference in cell growth was found between the Mitomut ESCs and WT ESCs (Fig. 3C). To further study the impacts on pluripotency, the embryoid body (EB) differentiation assay was performed and a decrease in EBs numbers differentiated from the Mitomut ESCs was identified, suggesting a defect in pluripotency. (Fig. 3E, F). What’s more, the Mitomut ESCs expressed a lower level of Rex1, a gene marker of pluripotent stem cells, and a higher level of Zscan4, a gene-marker of totipotent stem cells as compared with the wild-type ESCs, suggesting a modulation in the transformation and balance of pluripotency and totipotency (Fig. 3D). To investigate the impacts of mtDNA mutations on ESCs, we examined the metabolism of cells using Seahorse system. Compared to wild-type ESCs, ESCs with mtDNA mutants displayed a lower oxygen consumption rate (OCR) (Fig. 3G, H), suggesting a decrease in oxidative phosphorylation in energy production after the mtDNA mutation. It has been reported that the ESCs highly relied on glycolysis for energy production instead of oxidative phosphorylation (Ateghang et al., 2006; Zhou et al., 2012), which was in line with the finding that Mitomut ESCs harbored more totipotency genes expression (Troiano et al., 2020) (Fig. 3D–F). We also detected mitochondria-generated ROS, which showed an increase in Mitomut ESCs, which in further supported the modulation in metabolism after mtDNA mutation (Fig. 3I, J). In conclusion, our data suggested that mtDNA mutation impaired the pluripotency of ESCs by modulating their metabolic activity.
Fig. 3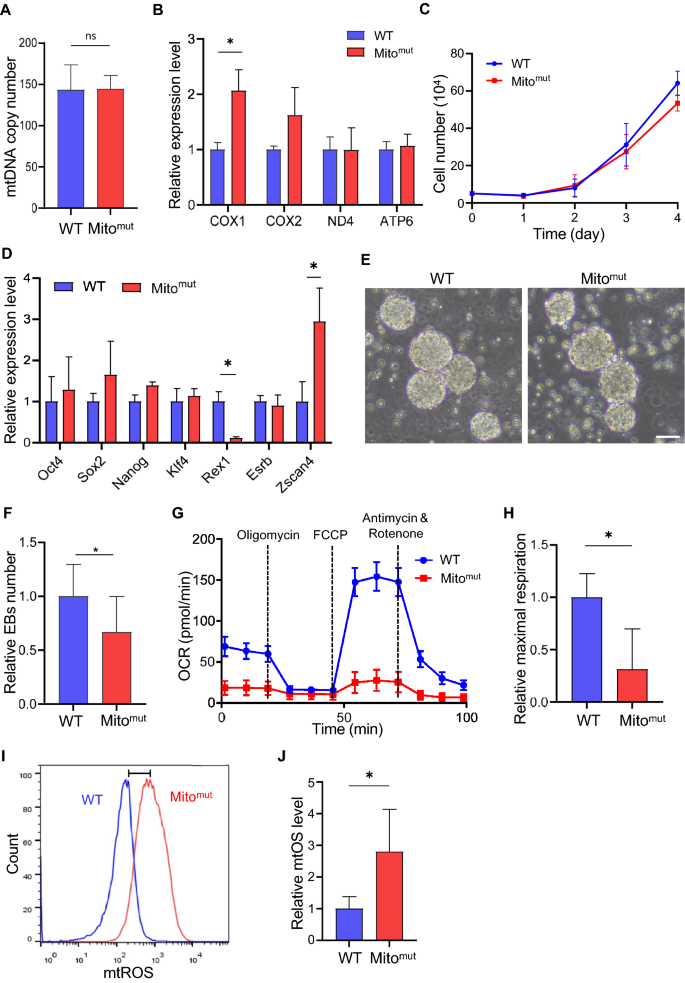
Defects in ESCs carrying mtDNA mutations. A The mtDNA copy number in identified ESCs carrying mtDNA mutations (Mitomut) and the wild-type ES cell line (WT). B Mitochondrial transcription in Mitomut and the WT ESCs. C The growth curve of Mitomut and WT ESCs. D The relative expression level of pluripotency marker genes detected by qPCR. E The EBs differentiated from Mitomut and WT ESCs. Scale bar: 50 μm. F The relative number of EBs differentiated from Mitomut and the WT ESCs. G Mitochondrial-stress test in Mitomut and the WT ESCs. H OCR value quantified from the mitochondrial-stress test in (G). I The mtROS level in Mitomut and the WT ESCs stained with MitoSOX Red. J The counting of mtROS in (I). Two-tailed unpaired t test were used and *p < 0.05
Developing an ESC model harboring mtDNA mutation without affecting genomic DNA is a novel approach for the study of mitochondrial diseases and development of new therapies. We also demonstrated the impacts on pluripotency and cell differentiation of ESCs made by mtDNA mutation and identified an impact on pluripotency with lower efficiency in differentiation (Fig. 3E, F). What’s more, the balance of pluripotency and totipotency may be a new pathway that regulated mitochondria in stem cells. The mtDNA mutation could regulate the pluripotency of ESCs through metabolism modulation of ESCs (Fig. 3F, G), which had a transformation in oxidative phosphorylation and glycolysis for energy production as reported (Ateghang et al., 2006; Zhou et al., 2012) (Fig. 3B). The ESCs harboring mtDNA mutation showed more characteristics of totipotency stem cells, which may also be regulated by the metabolic modulation such as glycolysis. In line with our previous report, the mitochondria-mediated metabolic modulation plays an important role not only in regulating oocytes aging (Yang et al., 2020), but also in the maintenance of ESC pluripotency. What’s more, the high level of ROS generation in ESCs as detected would further impact the genomic stability (Chaudhari et al., 2014; Maryanovich & Gross, 2013), which should be taken care of in mtDNA mutant diseases’ treatment.
Data availability
All data generated or analyzed during this study are included in this published article and its supplementary information files.
Abbreviations
DNA:
Deoxyribonucleic acid
mtDNA:
Mitochondrial deoxyribonucleic acid
WT:
Wild-type
Mut:
Mutant
ESC:
Embryonic stem cell
tRNA:
Transfer ribonucleic acid
rRNA:
Ribosomal ribonucleic acid
LHON:
Leber’s hereditary optic neuropathy
MELAS:
Mitochondrial encephalomyopathy, lactic acidosis, and stroke-like episodes;
EB:
Embryoid body
mESCs:
Mouse embryonic stem cells
GIBH:
Guangzhou Institutes of Biomedicine and Health, Chinese Academy of Sciences
PMSG:
Pregnant mare serum gonadotropin
hCG:
Human chorionic gonadotropin
MEF:
Mouse embryo fibroblasts
DMEM:
Dulbecco’s modified Eagle’s medium
NEAA:
Non-essential amino acid
PCR:
Polymerase chain reaction
qPCR:
Quantitative polymerase chain reaction
SNP:
Single-nucleotide polymorphism
mtROS:
Mitochondrial reactive oxygen species
LIF:
Leukemia inhibitory factor
FACS:
Fluorescence-activated cell sorting
OCR:
Oxygen consumption rate
FCCP:
Carbonyl cyanide-p-trifluoromethoxyphenylhydrazone
References
Al Rawi, S., Louvet-Vallée, S., Djeddi, A., Sachse, M., Culetto, E., Hajjar, C., Boyd, L., Legouis, R., & Galy, V. (2011). Postfertilization autophagy of sperm organelles prevents paternal mitochondrial DNA transmission. Science, 334(6059), 1144–1147. https://doi.org/10.1126/science.1211878
Anderson, S., Bankier, A. T., Barrell, B. G., de Bruijn, M. H., Coulson, A. R., Drouin, J., Eperon, I. C., Nierlich, D. P., Roe, B. A., Sanger, F., Schreier, P. H., Smith, A. J., Staden, R., & Young, I. G. (1981). Sequence and organization of the human mitochondrial genome. Nature, 290(5806), 457–465. https://doi.org/10.1038/290457a0
Ateghang, B., Wartenberg, M., Gassmann, M., & Sauer, H. (2006). Regulation of cardiotrophin-1 expression in mouse embryonic stem cells by HIF-1α and intracellular reactive oxygen species. Journal of Cell Science, 119(Pt 6), 1043–1052. https://doi.org/10.1242/jcs.02798
Bayona-Bafaluy, M. P., Movilla, N., Pérez-Martos, A., Fernández-Silva, P., & Enriquez, J. A. (2008). Functional genetic analysis of the mammalian mitochondrial DNA encoded peptides: A mutagenesis approach. Methods in Molecular Biology, 457, 379–390. https://doi.org/10.1007/978-1-59745-261-8_28
Bibb, M. J., Van Etten, R. A., Wright, C. T., Walberg, M. W., & Clayton, D. A. (1981). Sequence and gene organization of mouse mitochondrial DNA. Cell, 26(2 Pt 2), 167–180. https://doi.org/10.1016/0092-8674(81)90300-7
Chaudhari, P., Ye, Z., & Jang, Y. Y. (2014). Roles of reactive oxygen species in the fate of stem cells. Antioxidants Redox Signal., 20(12), 1881–1890. https://doi.org/10.1089/ars.2012.4963
Emperador, S., Lopez-Gallardo, E., Hernandez-Ainsa, C., Habbane, M., Montoya, J., Bayona-Bafaluy, M. P., & Ruiz-Pesini, E. (2019). Ketogenic treatment reduces the percentage of a LHON heteroplasmic mutation and increases mtDNA amount of a LHON homoplasmic mutation. Orphanet Journal of Rare Diseases, 14(1), 150. https://doi.org/10.1186/s13023-019-1128-z
Evans, M. J., & Kaufman, M. H. (1981). Establishment in culture of pluripotential cells from mouse embryos. Nature, 292(5819), 154–156. https://doi.org/10.1038/292154a0
Gomes, A. P., Price, N. L., Ling, A. J., Moslehi, J. J., Montgomery, M. K., Rajman, L., White, J. P., Teodoro, J. S., Wrann, C. D., Hubbard, B. P., Mercken, E. M., Palmeira, C. M., de Cabo, R., Rolo, A. P., Turner, N., Bell, E. L., & Sinclair, D. A. (2013). Declining NAD+ induces a pseudohypoxic state disrupting nuclear-mitochondrial communication during aging. Cell, 155(7), 1624–1638. https://doi.org/10.1016/j.cell.2013.11.037
Goto, Y., Nonaka, I., & Horai, S. (1990). A mutation in the tRNA Leu(UUR) gene associated with the MELAS subgroup of mitochondrial encephalomyopathies. Nature, 348(6302), 651–653. https://doi.org/10.1038/348651a0
Hance, N., Ekstrand, M. I., & Trifunovic, A. (2005). Mitochondrial DNA polymerase gamma is essential for mammalian embryogenesis. Human Molecular Genetics, 14(13), 1775–1783. https://doi.org/10.1093/hmg/ddi184
Iyer, S., Xiao, E., Alsayegh, K., Eroshenko, N., Riggs, M. J., Bennett, J. P., Jr., & Rao, R. R. (2012). Mitochondrial gene replacement in human pluripotent stem cell-derived neural progenitors. Gene Therapy, 19(5), 469–475. https://doi.org/10.1038/gt.2011.134
Ji, Y., Nie, Z., Meng, F., Hu, C., Chen, H., Jin, L., Chen, M., Zhang, M., Zhang, J., Liang, M., Wang, M., & Guan, M. X. (2021). Mechanistic insights into mitochondrial tRNAAla 3’-end metabolism deficiency. Journal of Biological Chemistry, 297(1), 100816. https://doi.org/10.1016/j.jbc.2021.100816
Kobayashi, Y., Momoi, M. Y., Tominaga, K., Momoi, T., Nihei, K., Yanagisawa, M., Kagawa, Y., & Ohta, S. (1990). A point mutation in the mitochondrial tRNALeu (UUR) gene in melas (mitochondrial myopathy, encephalopathy, lactic acidosis and stroke-like episodes). Biochemical and Biophysical Research Communications, 173(3), 816–822. https://doi.org/10.1016/s0006-291x(05)80860-5
Kujoth, G. C., Hiona, A., Pugh, T. D., Someya, S., Panzer, K., Wohlgemuth, S. E., Hofer, T., Seo, A. Y., Sullivan, R., Jobling, W. A., Morrow, J. D., Van Remmen, H., Sedivy, J. M., Yamasoba, T., Tanokura, M., Weindruch, R., Leeuwenburgh, C., & Prolla, T. A. (2005). Mitochondrial DNA mutations, oxidative stress, and apoptosis in mammalian aging. Science, 309(5733), 481–484. https://doi.org/10.1126/science.1112125
Martin, G. R. (1981). Isolation of a pluripotent cell line from early mouse embryos cultured in medium conditioned by teratocarcinoma stem cells. Proceedings of the National Academy of Sciences of the United States of America, 78(12), 7634–7638. https://doi.org/10.1073/pnas.78.12.7634
Maryanovich, M., & Gross, A. (2013). A ROS rheostat for cell fate regulation. Trends in Cell Biology, 23(3), 129–134. https://doi.org/10.1016/j.tcb.2012.09.007
Perry, E. A., Bennett, C. F., Luo, C., Balsa, E., Jedrychowski, M., O’Malley, K. E., Latorre-Muro, P., Ladley, R. P., Reda, K., Wright, P. M., Gygi, S. P., Myers, A. G., & Puigserver, P. (2021). Tetracyclines promote survival and fitness in mitochondrial disease models. Nature Metabolism, 3(1), 33–42. https://doi.org/10.1038/s42255-020-00334-y
Ropp, P. A., & Copeland, W. C. (1996). Cloning and characterization of the human mitochondrial DNA polymerase, DNA polymerase γ. Genomics, 36(3), 449–458. https://doi.org/10.1006/geno.1996.0490
Russell, O. M., Gorman, G. S., Lightowlers, R. N., & Turnbull, D. M. (2020). Mitochondrial diseases: Hope for the future. Cell, 181(1), 168–188. https://doi.org/10.1016/j.cell.2020.02.051
Santorelli, F. M., Shanske, S., Macaya, A., DeVivo, D. C., & DiMauro, S. (1993). The mutation at nt 8993 of mitochondrial DNA is a common cause of Leigh’s syndrome. Annals of Neurology, 34(6), 827–834. https://doi.org/10.1002/ana.410340612
Spelbrink, J. N., Toivonen, J. M., Hakkaart, G. A., Kurkela, J. M., Cooper, H. M., Lehtinen, S. K., Lecrenier, N., Back, J. W., Speijer, D., Foury, F., & Jacobs, H. T. (2000). In vivo functional analysis of the human mitochondrial DNA polymerase POLG expressed in cultured human cells. Journal of Biological Chemistry, 275(32), 24818–24828. https://doi.org/10.1074/jbc.M000559200
Tatuch, Y., Christodoulou, J., Feigenbaum, A., Clarke, J. T., Wherret, J., Smith, C., Rudd, N., Petrova-Benedict, R., & Robinson, B. H. (1992). Heteroplasmic mtDNA mutation (T→G) at 8993 can cause Leigh disease when the percentage of abnormal mtDNA is high. American Journal of Human Genetics, 50(4), 852–858.
Trifunovic, A., Wredenberg, A., Falkenberg, M., Spelbrink, J. N., Rovio, A. T., Bruder, C. E., Bohlooly-Y, M., Gidlöf, S., Oldfors, A., Wibom, R., Törnell, J., Jacobs, H. T., & Larsson, N. G. (2004). Premature ageing in mice expressing defective mitochondrial DNA polymerase. Nature, 429(6990), 417–423. https://doi.org/10.1038/nature02517
Troiano, A., Pacelli, C., Ruggieri, V., Scrima, R., Addeo, M., Agriesti, F., Lucci, V., Cavaliere, G., Mollica, M. P., Caterino, M., Ruoppolo, M., Paladino, S., Sarnataro, D., Visconte, F., Tucci, F., Lopriore, P., Calabrò, V., Capitanio, N., Piccoli, C., & Falco, G. (2020). ZSCAN4+ mouse embryonic stem cells have an oxidative and flexible metabolic profile. EMBO Reports, 21(6), e48942. https://doi.org/10.15252/embr.201948942
Vafai, S. B., & Mootha, V. K. (2012). Mitochondrial disorders as windows into an ancient organelle. Nature, 491(7424), 374–383. https://doi.org/10.1038/nature11707
van der Bliek, A. M., Sedensky, M. M., & Morgan, P. G. (2017). Cell biology of the mitochondrion. Genetics, 207(3), 843–871. https://doi.org/10.1534/genetics.117.300262
Wallace, D. C., Singh, G., Lott, M. T., Hodge, J. A., Schurr, T. G., Lezza, A. M., Elsas, L. J. 2nd., & Nikoskelainen, E. K. (1988). Mitochondrial DNA mutation associated with Leber’s hereditary optic neuropathy. Science, 242(4884), 1427-1430. https://doi.org/10.1126/science.3201231
Yang, L., Lin, X., Tang, H., Fan, Y., Zeng, S., Jia, L., Li, Y., Shi, Y., He, S., Wang, H., Hu, Z., Gong, X., Liang, X., Yang, Y., & Liu, X. (2020). Mitochondrial DNA mutation exacerbates female reproductive aging via impairment of the NADH/NAD+ redox. Aging Cell, 19(9), e13206. https://doi.org/10.1111/acel.13206
Zhou, W., Choi, M., Margineantu, D., Margaretha, L., Hesson, J., Cavanaugh, C., Blau, C. A., Horwitz, M. S., Hockenbery, D., Ware, C., & Ruohola-Baker, H. (2012). HIF1α induced switch from bivalent to exclusively glycolytic metabolism during ESC-to-EpiSC/hESC transition. EMBO Journal, 31(9), 2103–2116. https://doi.org/10.1038/emboj.2012.71
Zhou, Y., Long, Q., Wu, H., Li, W., Qi, J., Wu, Y., Xiang, G., Tang, H., Yang, L., Chen, K., Li, L., Bao, F., Li, H., Wang, Y., Li, M., & Liu, X. (2020). Topology-dependent, bifurcated mitochondrial quality control under starvation. Autophagy, 16(3), 562–574. https://doi.org/10.1080/15548627.2019.1634944
Funding
Our work was funded by the National Key Research and Development Program of China (2019YFA0904500, 2018YFA0107100, 2022YFA1103800), the Strategic Priority Research Program of the Chinese Academy of Sciences (XDA16030505), the National Natural Science Foundation Projects of China (92157202, 32025010, 92254301, 3221101041, 31900614, 31970709, 81901275, 32070729, 32100619, 32170747), the Key Research Program, CAS (ZDBS-ZRKJZ-TLC003, QYZDB-SSW-SMC001), the International Cooperation Program, CAS (154144KYSB20200006), CAS Project for Young Scientists in Basic Research (YSBR-075), Guangdong Province Science and Technology Program (2020B1212060052, 2020A1515011200, 2020A1515010919, 2020A1515011410, 2021A1515012513, 2021B1515020096, 2022A1515012616), and Guangzhou Science and Technology Program (202002030277, 202102020827, 202102080066), Open Research Program of Key Laboratory of Regenerative Biology, CAS (KLRB202107), and CAS Youth Innovation Promotion Association (to Y. W and K. C).
Author information
Juntao Qi and Qi Long equally contributed to this work.
Authors and Affiliations
CAS Key Laboratory of Regenerative Biology, Joint School of Life Sciences, Guangzhou, Institutes of Biomedicine and Health, Chinese Academy of Sciences, Guangzhou Medical University, Guangzhou, 510530, China
Juntao Qi, Qi Long, Yanshuang Zhou, Jian Zhang, Zifeng Ruan, Liang Yang, Yi Wu, Ge Xiang, Wei Li, Hao Wu, Shiwei Du & Xingguo Liu
Guangdong Provincial Key Laboratory of Stem Cell and Regenerative Medicine, China-New Zealand Joint Laboratory on Biomedicine and Health, CUHK-GIBH Joint Research Laboratory on Stem Cells and Regenerative Medicine, Institute for Stem Cell and Regeneration, Guangzhou Institutes of Biomedicine and Health, Chinese Academy of Sciences, Guangzhou, 510530, China
Juntao Qi, Qi Long, Yanshuang Zhou, Zifeng Ruan, Liang Yang, Yi Wu, Ge Xiang, Wei Li, Hao Wu, Shiwei Du & Xingguo Liu
University of Chinese Academy of Sciences, Beijing, 100049, China
Juntao Qi, Zifeng Ruan, Wei Li & Shiwei Du
School of Life Sciences, Sun Yat-sen University, Guangzhou, 510275, China
Yang Yuan
Centre for Regenerative Medicine and Health, Hong Kong Institute of Science and Innovation, Chinese Academy of Sciences, Hong Kong SAR, China
Xingguo Liu
Contributions
XL designed and supervised the project. JQ and QL designed and performed the experiments. QL and JQ wrote the manuscript. JZ helped with the animal experiments. All the authors have reviewed the manuscript.
Corresponding author
Correspondence to Xingguo Liu.
Ethics declarations
Conflict of interest
Author Xingguo Liu is a member of the Editorial Board for Genome Instability & Disease. The authors declare they have no financial interests.
Supplementary Information
Below is the link to the electronic supplementary material.
Supplementary file1 (DOCX 17 KB)
Rights and permissions
Springer Nature or its licensor (e.g. a society or other partner) holds exclusive rights to this article under a publishing agreement with the author(s) or other rightsholder(s); author self-archiving of the accepted manuscript version of this article is solely governed by the terms of such publishing agreement and applicable law.
About this article
Cite this article
Qi, J., Long, Q., Yuan, Y. et al. Mitochondrial DNA mutation affects the pluripotency of embryonic stem cells with metabolism modulation. GENOME INSTAB. DIS. (2022). https://doi.org/10.1007/s42764-022-00093-y
Received28 September 2022
Revised13 November 2022
Accepted20 November 2022
Published05 December 2022
DOIhttps://doi.org/10.1007/s42764-022-00093-y
Share this article
Anyone you share the following link with will be able to read this content:
Get shareable linkKeywords
Mitochondria
mtDNA
mtDNA mutation
Stem cell
ESC
Polg
用户登录
还没有账号?
立即注册