MutS and MutL sliding clamps in DNA mismatch repair
Review Article
Genome Instability & Disease (2022)
Abstract
DNA mismatch repair (MMR) corrects mispair nucleotides that arise from polymerase misincorporation. Highly conserved MutS (MSH) and MutL (MLH/PMS) homologs initiate MMR, and in higher eukaryotes act as DNA damage sensors that can trigger apoptosis. The MSH proteins recognize the mismatch nucleotides, whereas the MLH/PMS proteins mediate multiple protein–protein interactions associated with downstream MMR events including strand-specific incision and excision. Remarkably, the mechanics of the MSH and MLH/PMS proteins have been elusive for decades. Here we summarize and discuss recent biophysical studies of core MMR components, especially focusing on the unique conformations of MSH and MLH/PMS proteins that lead to the coordination of multiple repair events.
Introduction
Mispair DNA can result from nucleotide misincorporation during DNA replication, and is one of the basic types of DNA damage in cells (Fishel, 2015; Modrich, 1991). A highly conserved excision-resynthesis system named DNA mismatch repair (MMR) has been evolved in all three kingdoms of life to correct the mispair DNA and maintain genome stability (Fishel, 2015; Kolodner, 1996; Kunkel & Erie, 2005; Li, 2008). Defects in MMR genes, such as MutS (MSH) and MutL (MLH/PMS), increase the cellular mutation rates more than 100-fold, and in human are the causes of the common cancer predisposition Lynch syndrome or hereditary nonpolyposis colorectal cancer (LS/HNPCC) (Fishel, 2015; Hsieh, 2012; Lynch et al., 2015).
In addition to MMR, studies have found that MSH and MLH/PMS proteins were linked to DNA damage response in eukaryotes (Yoshioka et al., 2006). Consistently, MMR-deficient cells were shown to be resistant to cell death induced by alkylating agents, cisplatin, and ultraviolet radiation (O'Brien & Brown, 2006). Genetic studies have also suggested these MMR proteins participate in somatic hypermutation (SHM) and class switch recombination (CSR) in B lymphocytes, which are two critical processes during antibody diversification (Casali et al., 2006; Chahwan et al., 2011; Wilson et al., 2005). Moreover, recent findings have demonstrated that MSH and MLH/PMS proteins are involved in the modulation of cancer immunotherapy (Guan et al., 2021; Le et al., 2017; Lu et al., 2021), although the detailed mechanisms remain under intense investigations.
In this review, we mainly outline the molecular mechanisms of MMR and focus on the unique configurations of MutS and MutL homologs during MMR initiation. We discuss how the biophysical mechanics of these MMR proteins could result in a cascade of highly specific and efficient repair events, which highlights a mechanism of linking repair proteins onto DNA. The mechanics of MSH and MLH/PMS in DNA damage response, SHM, CSR or immunotherapy modulation are not considered here, however, seem unlikely to be elementally different.
MMR: an excision-resynthesis reaction
The genetic basis for MMR was established about 50 years ago, when a series of genes were found to be essential for gene conversions in bacteria and the defects in these genes elevated spontaneous mutation rates (Holliday, 1964; Miyake, 1960; Nevers & Spatz, 1975). In 1980s, several of these genes such as MutS, MutL, MutH and UvrD were linked to E. coli MMR (Radman et al., 1980). Methyl-directed DNA mismatch repair was then conceived (Carraway et al., 1987; Glickman & Radman, 1980; Laengle-Rouault et al., 1986; Lu et al., 1983) and the E. coli MMR was reconstituted in vitro using purified protein components including MutS, MutL, MutH, UvrD, single-stranded binding protein (SSB), polymerase III holoenzyme, exonuclease and DNA ligase (Lahue et al., 1987, 1989; Lu et al., 1983). While the MutS, MutL, MutH, UvrD, SSB and exonuclease have been demonstrated to execute the mismatch-dependent strand excision, gap resynthesis appears to be independent of the excision and is completed by the polymerase III holoenzyme as well as DNA ligase (Fig. 1) (Fishel, 2015).
Fig. 1
An illustration of the excision-resynthesis process of E. coli MMR. MutS protein recognizes the mismatch and recruits a MutL dimer onto the DNA. MutS and MutL proteins stimulate the activity of a MutH endonuclease to nick the unmethylated strand, as well as activate a UvrD helicase to unwind the dsDNA. SSB protects the nascent ssDNA and polymerase fills the gap
Full size imageMMR in E. coli has been extensively studied thus is well characterized. It has been established that E. coli MutS protein recognized the mismatch nucleotide to initiate MMR (Su & Modrich, 1986). Biochemical studies further showed that MutL physically interacted with MutS, forming a complex on mismatch DNA to recruit and activate MutH endonuclease (Grilley et al., 1989; Hall & Matson, 1999). MutH then recognized hemimethylated GATC sites which usually transiently occur during DNA replication, and specifically introduced scissions on the unmethylated strand that contains the misincorporated nucleotide (Ban & Yang, 1998a, b; Lee et al., 2005). These strand breaks may act as entry sites for UvrD helicase to unwind the double-stranded DNA (dsDNA) towards the mismatch (Cooper et al., 1993; Dao & Modrich, 1998; Grilley et al., 1993; Mechanic et al., 2000). Early studies proposed that SSB protected the nascent ssDNA tract and one of four redundant exonucleases excised the strand from the nick site to just past the mismatch (Burdett et al., 2001; Lahue et al., 1989; Viswanathan et al., 2001).
MMR components have also been identified in eukaryotes. In 1989, Kramer et al. demonstrated a high sequence similarity between S. cerevisiae Pms1 protein and E. coli MutL (Kramer et al., 1989). Several MutS and MutL homologs were determined in S. cerevisiae and human (Bronner et al., 1994; Drummond et al., 1995; Richard Fishel et al., 1993; Nicolaides et al., 1994; Palombo et al., 1995; Papadopoulos et al., 1994; Reenan & Kolodner, 1992). It was further reported that proliferating cell nuclear antigen (PCNA) (Johnson et al., 1996; Umar et al., 1996), exonuclease (Genschel et al., 2002; Schmutte et al., 1998, 2001; Tishkoff et al., 1998), DNA polymerase (Longley et al., 1997) and DNA ligases (Modrich & Lahue, 1996) were involved in a complete eukaryotic MMR reaction. The eukaryotic MMR excision has been considered to be similar as that of in E. coli, for example, it is bidirectional and requires a strand scission for initiation. However, MutH is not conserved outside of a subset of γ-proteobacteria family, and it appears that no helicase is involved in the eukaryotic MMR reaction (Putnam, 2016). Thus, the strand discrimination signals and MMR excision mechanics remain unclear in most organisms (Fishel, 2015; Kolodner, 2016).
Core MMR proteins: MutS and MutL homologs
The E. coli MutS usually forms a homodimer in solution and prefers binding to a DNA mismatch than a homoduplex (Mendillo et al., 2007). The crystal structure of a mismatch-bound MutS has been resolved by two groups in 2000 (Fig. 2a, left) (Lamers et al., 2000; Obmolova et al., 2000). The protein induces a 60° bending on the DNA backbone, and inserts a phenylalanine next to the nucleotide base to stabilize the bending (Lamers et al., 2000; Obmolova et al., 2000). Eukaryotic MutS homologs have very similar structures except function as heterodimers instead (Samir Acharya et al., 1996; Bowers et al., 1999; Drummond et al., 1995). All MutS homologs contain a highly conserved ATPase domain that belongs to the AAA+ superfamily, whereas the ATP binding and hydrolysis activities are essential for MMR (Gradia et al., 1997, 1999; Junop et al., 2001; Mendillo et al., 2005). In 1997, the ATPase activity of human MutS homolog MSH2-MSH6 was first shown to control the protein’s mismatch binding (Gradia et al., 1997). In the presence of ATP binding, all MutS homologs were later found to form ring-like structures that encircled the DNA in centre. These MutS ring-like structures could randomly diffuse along the DNA similar to a washer on a string, therefore they were originally named as MutS sliding clamps (Gradia et al., 1997, 1999). Nevertheless, the structure of an ATP-bound MutS has not been successfully determined until very recent (Fig. 2a, right) (Borsellini et al., 2022; Groothuizen et al., 2015). It was found that mismatch and ATP binding induced a conformational rearrangement of the MutS dimer, where tilting of MutS subunits across each other pushed DNA into a new channel (Groothuizen et al., 2015). This is the first direct observation showing how an ATP molecule induces the conformational switch of a MutS protein.
Fig. 2
Structures of core E. coli MMR proteins. a Left: Structure of a MutS bound to mismatch DNA (PDB ID: 1E3M). Right: Structure of an ATP-bound MutS sliding clamp (PDB ID: 7AIC). b Left: Structure of a nucleotide-free MutL dimer. N- and C-terminal structures are (PDB IDs: 1B63 and 1X9Z) joined by hypothetical flexible linkers. Right: Structure of an ATP-bound MutL sliding clamp
Full size imageThe MutL homologs also operate as homodimers or heterodimers, and usually contain three domains including the N-terminal ATPase domain, the C-terminal dimerization domain and a flexible linker domain that connects the N-terminal domain (NTD) and C-terminal domain (CTD) (Liu et al., 2018; London et al., 2021). The crystal structures of NTDs and CTDs from MutL and MLH/PMS have been separately determined (Fig. 2b, left) (1998b; Ban & Yang, 1998a; Ban et al., 1999; Guarné et al., 2001, 2004; Gueneau et al., 2013; Wu et al., 2015). However, any full-length structures of MutL homologs have not been available yet, probably because the intrinsically disordered linker domains are refractory to current structural analysis techniques. The NTDs of MutL and MLH/PMS are highly conserved among species and belong to the GHKL-superfamily (Gyrase, Hsp90, histidine kinase and MutL) ATPase (Ban & Yang, 1998a, b; Ban et al., 1999; Dutta & Inouye, 2000). Similar as MutS and MSH, the ATP binding and hydrolysis activities of MutL and MLH/PMS are also essential for MMR. Ban et al. showed that ATP binding induced the dimerization of MutL NTDs, whereas ATP hydrolysis was believed to disassemble the NTD dimer (Ban et al., 1999). The MutL NTDs contain a positively charged cleft (PCC), which was connected to an intrinsic DNA-binding activity detected under low ionic strength conditions (Ban et al., 1999; Guarné et al., 2004). Mutations of PCC residues have been shown to impair MMR both in vivo and in vitro, suggesting a functional PCC might be required for MMR (Arana et al., 2010; Groothuizen et al., 2015; Hall et al., 2001; Robertson et al., 2006). In contrast to NTDs, the sequences of CTDs of MutL and MLH/PMS are relatively divergent across species (Guarné et al., 2004). Nevertheless, all CTDs are capable of maintaining the homodimer/heterodimer associations during MMR (Gueneau et al., 2013). Interestingly, for the organisms that do not have a MutH homolog, MLH/PMS proteins contain a conserved endonuclease domain in their CTDs (Kadyrov et al., 2006, 2007; Pillon et al., 2010; Putnam, 2016). The dimer interactions and endonuclease activities of MLH/PMS have been shown to be crucial for MMR since mutations that disrupted the interactions/activities also caused a mutator phenotype in vivo (Aronshtam & Marinus, 1996; Chao et al., 2008; Guarné et al., 2004; Gueneau et al., 2013; Nishant et al., 2008; Reyes et al., 2020; Smith et al., 2013). The linker domains of MutL and MLH/PMS are variable in both sequence and length, which is consistent with their high degree of flexibility (Kim et al., 2019; London et al., 2021; Mardenborough et al., 2019). In the presence of ATP binding, a conformational rearrangement of MLH/PMS linker domains has been observed using atomic force microscopy, although it is still unclear how this rearrangement contributes to MMR (Sacho et al., 2008).
MMR models: debates lasted for more than two decades
To enhance the fidelity of DNA replication, MMR machineries must first recognize a mismatch, then discriminate between the parental and daughter DNA to exclusively initiate excision on the error-containing strand. It is generally considered that DNA strand breaks may serve as discrimination signals in both methyl-directed and methyl-independent MMR (Fishel, 2015; Kunkel & Erie, 2005; Li, 2008; Modrich & Lahue, 1996; Putnam, 2021). However, there are usually hundreds to thousands of base pairs between the mismatch and a strand break site (Fig. 1) (Fishel, 2015; Li, 2008). One obvious question is how the MMR machineries communicate between these two locations.
To answer this question, early biochemical analysis investigated the mechanics of MMR communications in E. coli, where MutS recognized the mismatch nucleotides and MutH endonuclease was activated at a distant hemi-methylated GATC site (Su & Modrich, 1986; Welsh et al., 1987). MutL was shown to play important roles in connecting mismatch recognition with strand-specific incision since it physically interacted with both MutS and MutH proteins (Acharya et al., 2003; Grilley et al., 1989; Hall & Matson, 1999). As described above, while both the MutS and MutL contain the ATPase activities that have been shown to be essential for MMR, a straightforward hydrolysis-dependent Translocation Model was initially proposed (Fig. 3a) (Allen et al., 1997; Iyer et al., 2006). In this model, MutS and MutL proteins form a stable complex at the mismatch, which further hydrolyze ATP and behave as motors to drive unidirectional translocation along the DNA (Allen et al., 1997). DNA from both sides of the mismatch are pulled through the complex until MutL encounters a MutH endonuclease at a hemi-methylated GATC site, a process that creates a DNA loop (Allen et al., 1997). After MutH introduces a strand break at the hemi-methylated site, UvrD helicase is then recruited for duplex DNA unwinding (Dao & Modrich, 1998). Although this model was consistent with most biochemical studies at that era, several issues remained. For example, how the directions of MutS-MutL translocations are managed away from the mismatch whereas UvrD helicase unwinds toward the mismatch? The ATPase activities of MutS and MutL are much weaker than a typical translocase such as a UvrD helicase, thus how would those weak ATPase activities sustain the efficient translocations along the DNA?
Fig. 3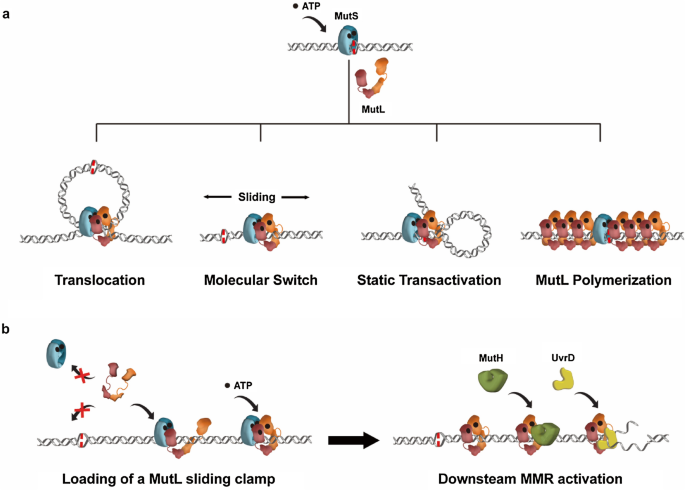
MMR models. a Historical models of MMR initiation. See text for detailed descriptions. b A molecular switch model of the complete E. coli MMR. Shape and color-coded MMR proteins are shown as indicated
Full size imageAnother Molecular Switch Model (also named as Sliding Clamp Model) was proposed in 1997 when Gradia et al. found the human MutS homolog MSH2-MSH6 functioned as a molecular switch (Fig. 3a) (Gradia et al., 1997). The initial concept suggested that ATP binding induced a conformational change in MSH2-MSH6, which further utilized thermal motions diffusing along DNA to activate downstream components. The configuration of an ATP-bound MSH2-MSH6 was later recognized as a ring-like sliding clamp on DNA (Gradia et al., 1999). Consistent with these findings, Acharya et al. showed the mismatch-provoked ATP binding induced the formation of multiple E. coli MutS sliding clamps on DNA (S. Acharya et al., 2003). These MutS sliding clamps no longer associated with mismatch nucleotide, instead, transited onto the adjacent duplex DNA and formed complexes with MutL to physically activate MutH endonuclease. In the Molecular Switch Model, ATP hydrolysis is used to recycle the MutS sliding clamps on DNA. Further genetic and biochemical studies strictly correlated the MutS/MSH protein’s ability to form a sliding clamp with its biological functions, strongly suggesting that the MutS/MSH sliding clamps are indispensable intermediates during MMR initiation (Graham et al., 2018; Hargreaves et al., 2010; Hess et al., 2002; Mendillo et al., 2005). While the MutS/MSH sliding clamps may operate as platforms for distant communications between a mismatch and a strand break site, a major puzzle in this model is how the MutL and MLH/PMS proteins coordinate downstream MMR events (Acharya et al., 2003).
The individual crystal structure of E. coli MMR proteins has been resolved in the 2000s, leading to the proposing of a Static Transactivation Model (Fig. 3a). Junop et al. (2001) reported that the mismatch recognition by MutS on one DNA molecule was capable of activating MutH to incise a GATC site located on another DNA without a mismatch. These findings suggested the formation of a static MutS-MutL complex on the mismatch induced DNA bending, which further enabled the complex to capture a strand break site. It was envisioned that MutS utilized its ATPase activity to verify mismatch binding and authorize MMR (Junop et al., 2001). Further studies showed both the NTD and CTD of MutL might be involved in DNA binding, resulting in a model where MutL mediated the DNA bending (Guarné et al., 2004). However, studies by Pluciennik and Modrich showed that placing a roadblock or a dsDNA break between a mismatch and a GATC site completely inhibited MMR, arguing in favour of a moving rather than a stationary mechanism (Pluciennik & Modrich, 2007).
A fourth MMR model was proposed based on a study of Saccharomyces cerevisiae fluorescent-tagged MMR proteins in situ (Fig. 3a). Hombauer et al. observed the mismatch recognition resulted in the formation of superstoichiometric Mlh1-Pms1 foci that were rarely co-localized with Msh2-Msh6 (Hombauer et al., 2011). Thus, Msh2-Msh6 was suggested to stably bind to the mismatch and induce polymerization of Mlh1-Pms1 along the DNA to reach a distant strand-discrimination site (Hombauer et al., 2011). Further in vitro studies have found the Thermus aquaticus MutS-MutL complex also contained more MutL than MutS, partially supporting for this MutL Polymerization Model (Qiu et al., 2015). Nevertheless, it is still unclear how the MutL or MLH/PMS is capable of forming MutS/MSH-dependent polymers along the mismatch DNA.
Discovery of MutL and MLH/PMS sliding clamps
As one might expect, using various DNA substrates as well as different reaction conditions could have led to the controversy of MMR model. More importantly, the intermediate steps of MMR reaction have not been clearly observed in most traditional biochemical studies. Thus, the exact mechanics of MutS and MutL homologs during MMR continued to be uncertain and appeared to be one of the most critical questions in the field. This has been ultimately solved by a series of biophysical studies from several groups using the state-of-art techniques, highlighting the importance of thermal motions in biology (Yang & Liu, 2021).
Greene’s lab first developed a DNA curtains-based assay to measure the behaviours of MMR proteins on DNA (Gorman et al., 2010a, 2010b; Greene et al., 2010). The DNA molecules were stretched by laminar flow and linked at one or both ends on a surface, whereas the MMR proteins were labelled with fluorescent quantum dots. DNA-binding events were acquired using a total internal reflection fluorescence (TIRF) microscope, which selectively illuminated a thin region of the specimen (~ 100—300 nm) to reduce fluorescence background. Both the yeast Msh2-Msh6 and Mlh1-Pms1 had been shown to perform one-dimensional diffusion along the dsDNA, although the physiological relevance of these behaviours was unclear at that time (). Using single-molecule fluorescence resonance energy transfer (smFRET) and single particle tracking, Jeong et al. (2011) and Cho et al. (2012) demonstrated that Thermus aquaticus MutS initially formed a searching clamp to scan dsDNA for mismatched nucleotides, whereas the mismatch recognition induced the formation of a long-live, ATP-bound MutS sliding clamp on DNA. The formations of eukaryotic ATP-bound MSH sliding clamps have also been observed in single-molecule level (Gorman et al., 2012; Honda et al., 2014), which further solidified the fact that the movements of MutS/MSH sliding clamps on DNA are driven by thermal motions other than energies of ATP hydrolysis. Later, the structure of a partial E. coli MutS-MutL complex that captured MutS in the sliding clamp conformation has been determined by Groothuizen et al. (2015), providing a direct evidence of the MutS sliding clamp formation. These findings have eventually established the biophysical progressions of MutS/MSH during MMR, which appears to support the Molecular Switch Model. Remarkably, most of these studies, if not all, have considered it was the sliding MutS/MSH that mediated the distant communications during MMR, where MutL or MLH/PMS functioned as a component of MMR machinery associated with the MutS/MSH sliding clamps.
In 2016, E. coli MutL has been found as a second ATP-bound sliding clamp on DNA during MMR (Fig. 2b, right) (Liu et al., 2016). It appeared that the MutS sliding clamp recruited a MutL dimer onto the DNA by initially forming a complex between MutS and a MutL NTD. ATP binding induced the dimerization of MutL NTDs, leading to the formation of a ring-like MutL clamp on the mismatched DNA. This long-live MutL clamp might dissociate from MutS and diffused randomly along the DNA, or oscillated as a sliding MutS–MutL complex with altered diffusion properties (Liu et al., 2016). Based on these observations, the MutL ring-like clamp configuration was named as “MutL sliding clamp”. Further studies have shown that only the MutL molecule in a ring-like clamp configuration was capable of recruiting MutH endonuclease and UvrD helicase onto DNA, suggesting that the MutL sliding clamp was playing a central role in downstream MMR activation events (Liu et al., 2016, 2019). In contrast, no MutL translocation, DNA looping formation or MutL polymerization was observed (Liu et al., 2016, 2019). Notably, the extension length of the mismatch DNA used in these studies was equivalent to 75% of its contour length (Liu et al., 2016), indicating the DNA molecules were not fully stretched thus unlikely prevented the DNA looping formation. The discovery of MutL sliding clamp has established that the MutL protein also functions as an ATP-dependent molecular switch (Liu et al., 2018). The principal role for ATP binding of MutL is to produce the ring-like configuration, whereas ATP hydrolysis appears to release the clamp from the DNA (Liu et al., 2016). Importantly, the MutS protein is absolutely required for the formation of a MutL sliding clamp, demonstrating the loading of this ring-like clamp is strictly mismatch-dependent. A similar cascade of sliding clamp progression has been observed with the human MSH2-MSH6 and MLH1-PMS2 heterodimers, although the roles of MLH1-PMS2 sliding clamp in eukaryotic MMR have to be further determined (London et al., 2021).
Followed by the discovery of MutL sliding clamp, two questions in E. coli MMR have been immediately raised: (1) Both an oscillating MutS-MutL complex and a MutL sliding clamp are capable of moving along the DNA, though they display distinct diffusion properties (Liu et al., 2016). Which molecule is responsible for the distant MMR communications? (2) The PCC of MutL and MLH/PMS has been suggested to induce DNA looping formation or protein polymerization via binding to dsDNA (Bradford et al., 2020; Hao et al., 2020; Ortega et al., 2021). MutL sliding clamp, however, has been found to diffuse much faster than a MutS sliding clamp and rarely associate with the DNA backbone (Liu et al., 2016, 2019). If any, what’s the role of PCC in MMR? A very recent study has demonstrated that MutL did not stably bind DNA under physiological ionic strength (Yang et al., 2022). However, in the presence of MutS and ATP, the PCC appeared to provide a crucial DNA docking environment for the loading of MutL sliding clamp (Yang et al., 2022). The PCC was also utilized by the MutL-MutH complex to search and incise hemi-methylated GATC sites, as well as employed by the MutL-UvrD complex to unwind the dsDNA. More interestingly, it was found that once a MutL sliding clamp had been loaded onto the DNA, there was no additional requirement for MutS during E. coli MMR incision/excision (Yang et al., 2022). These findings have established that the only role for a MutS-MutL complex is to load a MutL sliding clamp onto DNA, whereas the latter molecule solely activates downstream MMR events. These stable sliding clamps and the clamp-loader progressions strongly support the Molecular Switch Model, except the MutS sliding clamp in the original model is replaced by a cascade of MutS and MutL sliding clamps (Fig. 3b) (Acharya et al., 2003).
Conclusions and perspectives
The real-time single-molecule imaging technologies have ultimately visualized the complete strand-specific excision process in vitro to detail the MMR mechanism (Cho et al., 2012; Jeon et al., 2016; Jeong et al., 2011; Liu et al., 2016, 2019; Yang et al., 2022). In general, therefore, it seems like the mechanics and functions of MutL have been fully understood, at least in E. coli MMR. Nevertheless, the most intriguing unanswered questions still surround understanding the functions of MLH/PMS sliding clamps in eukaryotic MMR. Up to date no MutH or UvrD homologous protein has been found in eukaryotes and it is now well recognized that the eukaryotic 3′- and 5′-excision reactions require different MMR components in vitro. For example, the 3′-MMR excision requires the replicative processivity factor PCNA to activate the endonuclease activity of a MLH/PMS heterodimer, whereas an exonuclease is utilized in 5’-MMR (Goellner et al., 2015; Kadyrov et al., 2006, 2007; Zhang et al., 2005). It remains to be seen whether the Molecular Switch Model still stands in eukaryotic MMR. If yes, how the endonuclease activity of a MLH/PMS sliding clamp is stimulated by PCNA such that the MLH/PMS ring-like configuration exclusively incises the DNA strand containing the misincorporation (C. D. Putnam, 2021)? Moreover, while the exonuclease is absolutely required for MMR in vitro, it appears to be redundant in vivo (Hombauer et al., 2011; Smith et al., 2013). In contrast, it is possible of performing MLH/PMS-independent 5’-MMR in vitro while MMR in vivo strictly requires MLH/PMS proteins (Goellner et al., 2015; Jeon et al., 2016; Zhang et al., 2005). These results strongly suggest that the in vitro reconstituted MMR reactions in most historical studies might be different from MMR in vivo. One might image that a MLH/PMS sliding clamp is predominantly responsible for directing 5’-MMR excision in vivo. Further studies will be necessary to explore this idea.
Data availability
The authors confirm that the data supporting the findings of this study are available within the article.
References
Acharya, S., Foster, P. L., Brooks, P., & Fishel, R. (2003). The coordinated functions of the E. coli MutS and MutL proteins in mismatch repair. Molecular Cell, 12(1), 233–246. https://doi.org/10.1016/s1097-2765(03)00219-3
Acharya, S., Wilson, T., Gradia, S., Kane, M. F., Guerrette, S., Marsischky, G. T., Kolodner, R., & Fishel, R. (1996). hMSH2 forms specific mispair-binding complexes with hMSH3 and hMSH6. Proceedings of the National Academy of Sciences of the United States of America, 93(24), 13629–13634. https://doi.org/10.1073/pnas.93.24.13629
Allen, D. J., Makhov, A., Grilley, M., Taylor, J., Thresher, R., Modrich, P., & Griffith, J. D. (1997). MutS mediates heteroduplex loop formation by a translocation mechanism. The EMBO Journal, 16(14), 4467–4476. https://doi.org/10.1093/emboj/16.14.4467
Arana, M. E., Holmes, S. F., Fortune, J. M., Moon, A. F., Pedersen, L. C., & Kunkel, T. A. (2010). Functional residues on the surface of the N-terminal domain of yeast Pms1. DNA Repair (amst), 9(4), 448–457. https://doi.org/10.1016/j.dnarep.2010.01.010
Aronshtam, A., & Marinus, M. G. (1996). Dominant negative mutator mutations in the mutL gene of Escherichia coli. Nucleic Acids Research, 24(13), 2498–2504. https://doi.org/10.1093/nar/24.13.2498
Ban, C., Junop, M., & Yang, W. (1999). Transformation of MutL by ATP binding and hydrolysis: a switch in DNA mismatch repair. Cell, 97(1), 85–97. https://doi.org/10.1016/s0092-8674(00)80717-5
Ban, C., & Yang, W. (1998a). Structural basis for MutH activation in E. coli mismatch repair and relationship of MutH to restriction endonucleases. The EMBO Journal, 17(5), 1526–1534. https://doi.org/10.1093/emboj/17.5.1526
Ban, C., & Yang, W. (1998b). Crystal structure and ATPase activity of MutL: implications for DNA repair and mutagenesis. Cell. https://doi.org/10.1016/s0092-8674(00)81621-9
Borsellini, A., Kunetsky, V., Friedhoff, P., & Lamers, M. H. (2022). Cryogenic electron microscopy structures reveal how ATP and DNA binding in MutS coordinates sequential steps of DNA mismatch repair. Nature Structural & Molecular Biology, 29(1), 59–66. https://doi.org/10.1038/s41594-021-00707-1
Bowers, J., Sokolsky, T., Quach, T., & Alani, E. (1999). A mutation in the MSH6 subunit of the Saccharomyces cerevisiae MSH2-MSH6 complex disrupts mismatch recognition. Journal of Biological Chemistry, 274(23), 16115–16125. https://doi.org/10.1074/jbc.274.23.16115
Bradford, K. C., Wilkins, H., Hao, P., Li, Z. M., Wang, B., Burke, D., Wu, D., Smith, A. E., Spaller, L., Du, C., Gauer, J. W., Chan, E., Hsieh, P., Weninger, K. R., & Erie, D. A. (2020). Dynamic human MutSalpha-MutLalpha complexes compact mismatched DNA. Proceedings of the National Academy of Sciences of the United States of America. https://doi.org/10.1073/pnas.1918519117
Bronner, C. E., Baker, S. M., Morrison, P. T., Warren, G., Smith, L. G., Lescoe, M. K., Kane, M., Earabino, C., Lipford, J., & Lindblom, A. (1994). Mutation in the DNA mismatch repair gene homologue hMLH 1 is associated with hereditary non-polyposis colon cancer. Nature, 368(6468), 258–261. https://doi.org/10.1038/368258a0
Burdett, V., Baitinger, C., Viswanathan, M., Lovett, S. T., & Modrich, P. (2001). In vivo requirement for RecJ, ExoVII, ExoI, and ExoX in methyl-directed mismatch repair. Proceedings of the National Academy of Sciences of the United States of America, 98(12), 6765–6770. https://doi.org/10.1073/pnas.121183298
Carraway, M., Youderian, P., & Marinus, M. G. (1987). Spontaneous mutations occur near dam recognition sites in a dam-Escherichia coli host. Genetics, 116(3), 343–347. https://doi.org/10.1093/genetics/116.3.343
Casali, P., Pal, Z., Xu, Z., & Zan, H. (2006). DNA repair in antibody somatic hypermutation. Trends in Immunology, 27(7), 313–321. https://doi.org/10.1016/j.it.2006.05.001
Chahwan, R., Edelmann, W., Scharff, M. D., & Roa, S. (2011). Mismatch-mediated error prone repair at the immunoglobulin genes. Biomedicine Pharmacotherapy, 65(8), 529–536. https://doi.org/10.1016/j.biopha.2011.09.001
Chao, E. C., Velasquez, J. L., Witherspoon, M. S., Rozek, L. S., Peel, D., Ng, P., Gruber, S. B., Watson, P., Rennert, G., Anton-Culver, H., Lynch, H., & Lipkin, S. M. (2008). Accurate classification of MLH1/MSH2 missense variants with multivariate analysis of protein polymorphisms–mismatch repair (MAPP-MMR). Human Mutation, 29(6), 852–860. https://doi.org/10.1002/humu.20735
Cho, W. K., Jeong, C., Kim, D., Chang, M., Song, K. M., Hanne, J., Ban, C., Fishel, R., & Lee, J. B. (2012). ATP alters the diffusion mechanics of MutS on mismatched DNA. Structure, 20(7), 1264–1274. https://doi.org/10.1016/j.str.2012.04.017
Cooper, D. L., Lahue, R. S., & Modrich, P. (1993). Methyl-directed mismatch repair is bidirectional. The Journal of Biological Chemistry, 268(16), 11823–11829. https://doi.org/10.1016/S0021-9258(19)50274-5
Dao, V., & Modrich, P. (1998). Mismatch-, MutS-, MutL-, and helicase II-dependent unwinding from the single-strand break of an incised heteroduplex. The Journal of Biological Chemistry, 273(15), 9202–9207. https://doi.org/10.1074/jbc.273.15.9202
Drummond, J. T., Li, G. M., Longley, M. J., & Modrich, P. (1995). Isolation of an hMSH2-p160 heterodimer that restores DNA mismatch repair to tumor cells. Science, 268(5219), 1909–1912. https://doi.org/10.1126/science.7604264
Dutta, R., & Inouye, M. (2000). GHKL, an emergent ATPase/kinase superfamily. Trends in Biochemical Sciences, 25(1), 24–28. https://doi.org/10.1016/s0968-0004(99)01503-0
Fishel, R. (2015). Mismatch repair. The Journal of Biological Chemistry, 290(44), 26395–26403. https://doi.org/10.1074/jbc.R115.660142
Fishel, R., Lescoe, M. K., Rao, M. R., Copeland, N. G., Jenkins, N. A., Garber, J., Kane, M., & Kolodner, R. (1993). The human mutator gene homolog MSH2 and its association with hereditary nonpolyposis colon cancer. Cell, 75(5), 1027–1038. https://doi.org/10.1016/0092-8674(93)90546-3
Genschel, J., Bazemore, L. R., & Modrich, P. (2002). Human exonuclease I is required for 5’ and 3’ mismatch repair. The Journal of Biological Chemistry, 277(15), 13302–13311. https://doi.org/10.1074/jbc.M111854200
Glickman, B. W., & Radman, M. (1980). Escherichia coli mutator mutants deficient in methylation-instructed DNA mismatch correction. Proceedings of the National Academy of Sciences of the United States of America, 77(2), 1063–1067. https://doi.org/10.1073/pnas.77.2.1063
Goellner, E. M., Putnam, C. D., & Kolodner, R. D. (2015). Exonuclease 1-dependent and independent mismatch repair. DNA Repair (amst), 32, 24–32. https://doi.org/10.1016/j.dnarep.2015.04.010
Gorman, J., Chowdhury, A., Surtees, J. A., Shimada, J., Reichman, D. R., Alani, E., & Greene, E. C. (2007). Dynamic basis for one-dimensional DNA scanning by the mismatch repair complex Msh2-Msh6. Molecular Cell, 28(3), 359–370. https://doi.org/10.1016/j.molcel.2007.09.008
Gorman, J., Fazio, T., Wang, F., Wind, S., & Greene, E. C. (2010a). Nanofabricated racks of aligned and anchored DNA substrates for single-molecule imaging. Langmuir: THe ACS Journal of Surfaces and Colloids, 26(2), 1372–1379. https://doi.org/10.1021/la902443e
Gorman, J., Plys, A. J., Visnapuu, M. L., Alani, E., & Greene, E. C. (2010b). Visualizing one-dimensional diffusion of eukaryotic DNA repair factors along a chromatin lattice. Nature Structural & Molecular Biology, 17(8), 932–938. https://doi.org/10.1038/nsmb.1858
Gorman, J., Wang, F., Redding, S., Plys, A. J., Fazio, T., Wind, S., Alani, E. E., & Greene, E. C. (2012). Single-molecule imaging reveals target-search mechanisms during DNA mismatch repair. Proceedings of the National Academy of Sciences of the United States of America, 109(45), E3074-3083. https://doi.org/10.1073/pnas.1211364109
Gradia, S., Acharya, S., & Fishel, R. (1997). The human mismatch recognition complex hMSH2-hMSH6 functions as a novel molecular switch. Cell, 91(7), 995–1005. https://doi.org/10.1016/s0092-8674(00)80490-0
Gradia, S., Subramanian, D., Wilson, T., Acharya, S., Makhov, A., Griffith, J., & Fishel, R. (1999). hMSH2-hMSH6 forms a hydrolysis-independent sliding clamp on mismatched DNA. Molecular Cell, 3(2), 255–261. https://doi.org/10.1016/s1097-2765(00)80316-0
Graham, W. J. T., Putnam, C. D., & Kolodner, R. D. (2018). The properties of Msh2-Msh6 ATP binding mutants suggest a signal amplification mechanism in DNA mismatch repair. The Journal of Biological Chemistry, 293(47), 18055–18070. https://doi.org/10.1074/jbc.RA118.005439
Greene, E. C., Wind, S., Fazio, T., Gorman, J., & Visnapuu, M. L. (2010). DNA curtains for high-throughput single-molecule optical imaging. Methods in enzymology (Vol. 472, pp. 293–315). Elsevier. https://doi.org/10.1016/S0076-6879(10)72006-1
Grilley, M., Griffith, J., & Modrich, P. (1993). Bidirectional excision in methyl-directed mismatch repair. The Journal of Biological Chemistry, 268(16), 11830–11837. https://doi.org/10.1016/S0021-9258(19)50275-7
Grilley, M., Welsh, K. M., Su, S. S., & Modrich, P. (1989). Isolation and characterization of the Escherichia coli mutL gene product. The Journal of Biological Chemistry, 264(2), 1000–1004. https://doi.org/10.1016/S0021-9258(19)85043-3
Groothuizen, F. S., Winkler, I., Cristóvão, M., Fish, A., Winterwerp, H. H., Reumer, A., Marx, A. D., Hermans, N., Nicholls, R. A., Murshudov, G. N., Lebbink, J. H., Friedhoff, P., & Sixma, T. K. (2015). MutS/MutL crystal structure reveals that the MutS sliding clamp loads MutL onto DNA. eLife, 4, e06744. https://doi.org/10.7554/eLife.06744
Guan, J., Lu, C., Jin, Q., Lu, H., Chen, X., Tian, L., Zhang, Y., Ortega, J., Zhang, J., Siteni, S., Chen, M., Gu, L., Shay, J. W., Davis, A. J., Chen, Z. J., Fu, Y. X., & Li, G. M. (2021). MLH1 deficiency-triggered DNA hyperexcision by exonuclease 1 activates the cGAS-STING pathway. Cancer Cell, 39(1), 109-121e105. https://doi.org/10.1016/j.ccell.2020.11.004
Guarné, A., Junop, M. S., & Yang, W. (2001). Structure and function of the N-terminal 40 kDa fragment of human PMS2: A monomeric GHL ATPase. The EMBO Journal, 20(19), 5521–5531. https://doi.org/10.1093/emboj/20.19.5521
Guarné, A., Ramon-Maiques, S., Wolff, E. M., Ghirlando, R., Hu, X., Miller, J. H., & Yang, W. (2004). Structure of the MutL C-terminal domain: A model of intact MutL and its roles in mismatch repair. The EMBO Journal, 23(21), 4134–4145. https://doi.org/10.1038/sj.emboj.7600412
Gueneau, E., Dherin, C., Legrand, P., Tellier-Lebegue, C., Gilquin, B., Bonnesoeur, P., Londino, F., Quemener, C., Le Du, M. H., Márquez, J. A., Moutiez, M., Gondry, M., Boiteux, S., & Charbonnier, J. B. (2013). Structure of the MutLα C-terminal domain reveals how Mlh1 contributes to Pms1 endonuclease site. Nature Structural & Molecular Biology, 20(4), 461–468. https://doi.org/10.1038/nsmb.2511
Hall, M. C., & Matson, S. W. (1999). The Escherichia coli MutL protein physically interacts with MutH and stimulates the MutH-associated endonuclease activity. The Journal of Biological Chemistry, 274(3), 1306–1312. https://doi.org/10.1074/jbc.274.3.1306
Hall, M. C., Wang, H., Erie, D. A., & Kunkel, T. A. (2001). High affinity cooperative DNA binding by the yeast Mlh1-Pms1 heterodimer. The Journal of Biological Chemistry, 312(4), 637–647. https://doi.org/10.1006/jmbi.2001.4958
Hao, P., LeBlanc, S. J., Case, B. C., Elston, T. C., Hingorani, M. M., Erie, D. A., & Weninger, K. R. (2020). Recurrent mismatch binding by MutS mobile clamps on DNA localizes repair complexes nearby. Proceedings of the National Academy of Sciences of the United States of America. https://doi.org/10.1073/pnas.1918517117
Hargreaves, V. V., Shell, S. S., Mazur, D. J., Hess, M. T., & Kolodner, R. D. (2010). Interaction between the Msh2 and Msh6 nucleotide-binding sites in the Saccharomyces cerevisiae Msh2-Msh6 complex. The Journal of Biological Chemistry, 285(12), 9301–9310. https://doi.org/10.1074/jbc.M109.096388
Hess, M. T., Gupta, R. D., & Kolodner, R. D. (2002). Dominant Saccharomyces cerevisiae msh6 mutations cause increased mispair binding and decreased dissociation from mispairs by Msh2-Msh6 in the presence of ATP. The Journal of Biological Chemistry, 277(28), 25545–25553. https://doi.org/10.1074/jbc.M202282200
Holliday, R. (1964). A mechanism for gene conversion in fungi. Genetics Research, 5(2), 282–304. https://doi.org/10.1017/S0016672308009476
Hombauer, H., Campbell, C. S., Smith, C. E., Desai, A., & Kolodner, R. D. (2011). Visualization of eukaryotic DNA mismatch repair reveals distinct recognition and repair intermediates. Cell, 147(5), 1040–1053. https://doi.org/10.1016/j.cell.2011.10.025
Honda, M., Okuno, Y., Hengel, S. R., Martín-López, J. V., Cook, C. P., Amunugama, R., Soukup, R. J., Subramanyam, S., Fishel, R., & Spies, M. (2014). Mismatch repair protein hMSH2–hMSH6 recognizes mismatches and forms sliding clamps within a D-loop recombination intermediate. Proceedings of the National Academy of Sciences of the United States of America, 111(3), E316–E325. https://doi.org/10.1073/pnas.1312988111
Hsieh, P. (2012). DNA mismatch repair: Dr. Jekyll and Mr. Hyde? Molecular cell, 47(5), 665–666. https://doi.org/10.1016/j.molcel.2012.08.020
Iyer, R. R., Pluciennik, A., Burdett, V., & Modrich, P. L. (2006). DNA mismatch repair: Functions and mechanisms. Chemical Reviews, 106(2), 302–323. https://doi.org/10.1021/cr0404794
Jeon, Y., Kim, D., Martín-López, J. V., Lee, R., Oh, J., Hanne, J., Fishel, R., & Lee, J. B. (2016). Dynamic control of strand excision during human DNA mismatch repair. Proceedings of the National Academy of Sciences of the United States of America, 113(12), 3281–3286. https://doi.org/10.1073/pnas.1523748113
Jeong, C., Cho, W. K., Song, K. M., Cook, C., Yoon, T. Y., Ban, C., Fishel, R., & Lee, J. B. (2011). MutS switches between two fundamentally distinct clamps during mismatch repair. Nature Structural & Molecular Biology, 18(3), 379–385. https://doi.org/10.1038/nsmb.2009
Johnson, R. E., Kovvali, G. K., Guzder, S. N., Amin, N. S., Holm, C., Habraken, Y., Sung, P., Prakash, L., & Prakash, S. (1996). Evidence for involvement of yeast proliferating cell nuclear antigen in DNA mismatch repair. The Journal of Biological Chemistry, 271(45), 27987–27990. https://doi.org/10.1074/jbc.271.45.27987
Junop, M. S., Obmolova, G., Rausch, K., Hsieh, P., & Yang, W. (2001). Composite active site of an ABC ATPase: MutS uses ATP to verify mismatch recognition and authorize DNA repair. Molecular Cell, 7(1), 1–12. https://doi.org/10.1016/s1097-2765(01)00149-6
Kadyrov, F. A., Dzantiev, L., Constantin, N., & Modrich, P. (2006). Endonucleolytic function of MutLalpha in human mismatch repair. Cell, 126(2), 297–308. https://doi.org/10.1016/j.cell.2006.05.039
Kadyrov, F. A., Holmes, S. F., Arana, M. E., Lukianova, O. A., O’Donnell, M., Kunkel, T. A., & Modrich, P. (2007). Saccharomyces cerevisiae MutLα is a mismatch repair endonuclease. The Journal of Biological Chemistry, 282(51), 37181–37190. https://doi.org/10.1074/jbc.M707617200
Kim, Y., Furman, C. M., Manhart, C. M., Alani, E., & Finkelstein, I. J. (2019). Intrinsically disordered regions regulate both catalytic and non-catalytic activities of the MutLα mismatch repair complex. Nucleic Acids Research, 47(4), 1823–1835. https://doi.org/10.1093/nar/gky1244
Kolodner, R. (1996). Biochemistry and genetics of eukaryotic mismatch repair. Genes & Development, 10(12), 1433–1442. https://doi.org/10.1101/gad.10.12.1433
Kolodner, R. D. (2016). A personal historical view of DNA mismatch repair with an emphasis on eukaryotic DNA mismatch repair. DNA Repair, 38, 3–13. https://doi.org/10.1016/j.dnarep.2015.11.009
Kramer, W., Kramer, B., Williamson, M. S., & Fogel, S. (1989). Cloning and nucleotide sequence of DNA mismatch repair gene PMS1 from Saccharomyces cerevisiae: homology of PMS1 to procaryotic MutL and HexB. Journal of Bacteriology, 171(10), 5339–5346. https://doi.org/10.1128/jb.171.10.5339-5346.1989
Kunkel, T. A., & Erie, D. A. (2005). DNA mismatch repair. Annual Review of Biochemistry, 74(1), 681–710. https://doi.org/10.1146/annurev.biochem.74.082803.133243
Laengle-Rouault, F., Maenhaut-Michel, G., & Radman, M. (1986). GATC sequence and mismatch repair in Escherichia coli. The EMBO Journal, 5(8), 2009–2013. https://doi.org/10.1002/j.1460-2075.1986.tb04457.x
Lahue, R., Au, K., & Modrich, P. (1989). DNA mismatch correction in a defined system. Science, 245(4914), 160–164. https://doi.org/10.1126/science.2665076
Lahue, R. S., Su, S. S., & Modrich, P. (1987). Requirement for d(GATC) sequences in Escherichia coli mutHLS mismatch correction. Proceedings of the National Academy of Sciences of the United States of America, 84(6), 1482–1486. https://doi.org/10.1073/pnas.84.6.1482
Lamers, M. H., Perrakis, A., Enzlin, J. H., Winterwerp, H. H. K., De Wind, N., & Sixma, T. K. (2000). The crystal structure of DNA mismatch repair protein MutS binding to a G·T mismatch. Nature, 407(6805), 711–717. https://doi.org/10.1038/35037523
Le, D. T., Durham, J. N., Smith, K. N., Wang, H., Bartlett, B. R., Aulakh, L. K., Lu, S., Kemberling, H., Wilt, C., Luber, B. S., Wong, F., Azad, N. S., Rucki, A. A., Laheru, D., Donehower, R., Zaheer, A., Fisher, G. A., Crocenzi, T. S., Lee, J. J., … Diaz, L. A., Jr. (2017). Mismatch repair deficiency predicts response of solid tumors to PD-1 blockade. Science, 357(6349), 409–413. https://doi.org/10.1126/science.aan6733
Lee, J. Y., Chang, J., Joseph, N., Ghirlando, R., Rao, D. N., & Yang, W. (2005). MutH complexed with hemi-and unmethylated DNAs: coupling base recognition and DNA cleavage. Molecular Cell, 20(1), 155–166. https://doi.org/10.1016/j.molcel.2005.08.019
Li, G. M. (2008). Mechanisms and functions of DNA mismatch repair. Cell Research, 18(1), 85–98. https://doi.org/10.1038/cr.2007.115
Liu, J., Hanne, J., Britton, B. M., Bennett, J., Kim, D., Lee, J. B., & Fishel, R. (2016). Cascading MutS and MutL sliding clamps control DNA diffusion to activate mismatch repair. Nature, 539(7630), 583–587. https://doi.org/10.1038/nature20562
Liu, J., Lee, J. B., & Fishel, R. (2018). Stochastic processes and component plasticity governing DNA mismatch repair. Journal of Molecular Biology, 430(22), 4456–4468. https://doi.org/10.1016/j.jmb.2018.05.039
Liu, J., Lee, R., Britton, B. M., London, J. A., Yang, K., Hanne, J., Lee, J. B., & Fishel, R. (2019). MutL sliding clamps coordinate exonuclease-independent Escherichia coli mismatch repair. Nature Communications, 10(1), 5294. https://doi.org/10.1038/s41467-019-13191-5
London, J., Martin-Lopez, J., Yang, I., Liu, J., Lee, J. B., & Fishel, R. (2021). Linker domain function predicts pathogenic MLH1 missense variants. Proceedings of the National Academy of Sciences of the United States of America. https://doi.org/10.1073/pnas.2019215118
Longley, M. J., Pierce, A. J., & Modrich, P. (1997). DNA polymerase delta is required for human mismatch repair in vitro. The Journal of Biological Chemistry, 272(16), 10917–10921. https://doi.org/10.1074/jbc.272.16.10917
Lu, A.-L., Clark, S., & Modrich, P. (1983). Methyl-directed repair of DNA base-pair mismatches in vitro. DNA Repair (amst), 80(15), 4639–4643. https://doi.org/10.1073/pnas.80.15.4639
Lu, C., Guan, J., Lu, S., Jin, Q., Rousseau, B., Lu, T., Stephens, D., Zhang, H., Zhu, J., Yang, M., Ren, Z., Liang, Y., Liu, Z., Han, C., Liu, L., Cao, X., Zhang, A., Qiao, J., Batten, K., … Fu, Y. X. (2021). DNA sensing in mismatch repair-deficient tumor cells is essential for anti-tumor immunity. Cancer Cell, 39(1), 96-108.e106. https://doi.org/10.1016/j.ccell.2020.11.006
Lynch, H. T., Snyder, C. L., Shaw, T. G., Heinen, C. D., & Hitchins, M. P. (2015). Milestones of Lynch syndrome: 1895–2015. Nature Reviews Cancer, 15(3), 181–194. https://doi.org/10.1038/nrc3878
Mardenborough, Y. S. N., Nitsenko, K., Laffeber, C., Duboc, C., Sahin, E., Quessada-Vial, A., Winterwerp, H. H. K., Sixma, T. K., Kanaar, R., Friedhoff, P., Strick, T. R., & Lebbink, J. H. G. (2019). The unstructured linker arms of MutL enable GATC site incision beyond roadblocks during initiation of DNA mismatch repair. Nucleic Acids Research, 47(22), 11667–11680. https://doi.org/10.1093/nar/gkz834
Mechanic, L. E., Frankel, B. A., & Matson, S. W. (2000). Escherichia coli MutL loads DNA helicase II onto DNA. The Journal of Biological Chemistry, 275(49), 38337–38346. https://doi.org/10.1074/jbc.M006268200
Mendillo, M. L., Mazur, D. J., & Kolodner, R. D. (2005). Analysis of the interaction between the Saccharomyces cerevisiae MSH2-MSH6 and MLH1-PMS1 complexes with DNA using a reversible DNA end-blocking system. The Journal of Biological Chemistry, 280(23), 22245–22257. https://doi.org/10.1074/jbc.M407545200
Mendillo, M. L., Putnam, C. D., & Kolodner, R. D. (2007). Escherichia coli MutS tetramerization domain structure reveals that stable dimers but not tetramers are essential for DNA mismatch repair in vivo. The Journal of Biological Chemistry, 282(22), 16345–16354. https://doi.org/10.1074/jbc.M700858200
Miyake, T. (1960). Mutator factor in Salmonella typhimurium. Genetics, 45(1), 11. https://doi.org/10.1093/genetics/45.1.11
Modrich, P. (1991). Mechanisms and biological effects of mismatch repair. Annual Review of Genetics, 25(1), 229–253. https://doi.org/10.1146/annurev.ge.25.120191.001305
Modrich, P., & Lahue, R. (1996). Mismatch repair in replication fidelity, genetic recombination, and cancer biology. Annual Review of Biochemistry, 65(1), 101–133. https://doi.org/10.1146/annurev.bi.65.070196.000533
Nevers, P., & Spatz, H. C. (1975). Escherichia coli mutants uvr D and uvr E deficient in gene conversion of λ-heteroduplexes. Molecular & General Genetics, 139(3), 233–243. https://doi.org/10.1007/BF00268974
Nicolaides, N. C., Papadopoulos, N., Liu, B., Wei, Y. F., Carter, K. C., Ruben, S. M., Rosen, C. A., Haseltine, W. A., Fleischmann, R. D., & Fraser, C. M. (1994). Mutations of two P/WS homologues in hereditary nonpolyposis colon cancer. Nature, 371(6492), 75–80. https://doi.org/10.1038/371075a0
Nishant, K., Plys, A. J., & Alani, E. (2008). A mutation in the putative MLH3 endonuclease domain confers a defect in both mismatch repair and meiosis in Saccharomyces cerevisiae. Genetics, 179(2), 747–755. https://doi.org/10.1534/genetics.108.086645
Obmolova, G., Ban, C., Hsieh, P., & Yang, W. (2000). Crystal structures of mismatch repair protein MutS and its complex with a substrate DNA. Nature, 407(6805), 703–710. https://doi.org/10.1038/35037509
O’Brien, V., & Brown, R. (2006). Signalling cell cycle arrest and cell death through the MMR system. Carcinogenesis, 27(4), 682–692. https://doi.org/10.1093/carcin/bgi298
Ortega, J., Lee, G. S., Gu, L., Yang, W., & Li, G. M. (2021). Mispair-bound human MutS–MutL complex triggers DNA incisions and activates mismatch repair. Cell Research. https://doi.org/10.1038/s41422-021-00468-y
Palombo, F., Gallinari, P., Iaccarino, I., Lettieri, T., Hughes, M., D’Arrigo, A., Truong, O., Hsuan, J. J., & Jiricny, J. (1995). GTBP, a 160-kilodalton protein essential for mismatch-binding activity in human cells. Science, 268(5219), 1912–1914. https://doi.org/10.1126/science.7604265
Papadopoulos, N., Nicolaides, N. C., Wei, Y. F., Ruben, S. M., Carter, K. C., Rosen, C. A., Haseltine, W. A., Fleischmann, R. D., Fraser, C. M., & Adams, M. D. (1994). Mutation of a mutL homolog in hereditary colon cancer. Science, 263(5153), 1625–1629. https://doi.org/10.1126/science.8128251
Pillon, M. C., Lorenowicz, J. J., Uckelmann, M., Klocko, A. D., Mitchell, R. R., Chung, Y. S., Modrich, P., Walker, G. C., Simmons, L. A., Friedhoff, P., & Guarné, A. (2010). Structure of the endonuclease domain of MutL: unlicensed to cut. Molecular Cell, 39(1), 145–151. https://doi.org/10.1016/j.molcel.2010.06.027
Pluciennik, A., & Modrich, P. (2007). Protein roadblocks and helix discontinuities are barriers to the initiation of mismatch repair. Proceedings of the National Academy of Sciences of the United States of America, 104(31), 12709–12713. https://doi.org/10.1073/pnas.0705129104
Putnam, C. D. (2016). Evolution of the methyl directed mismatch repair system in Escherichia coli. DNA Repair, 38, 32–41. https://doi.org/10.1016/j.dnarep.2015.11.016
Putnam, C. D. (2021). Strand discrimination in DNA mismatch repair. DNA Repair (amst), 105, 103161. https://doi.org/10.1016/j.dnarep.2021.103161
Qiu, R., Sakato, M., Sacho, E. J., Wilkins, H., Zhang, X., Modrich, P., Hingorani, M. M., Erie, D. A., & Weninger, K. R. (2015). MutL traps MutS at a DNA mismatch. Proceedings of the National Academy of Sciences of the United States of America, 112(35), 10914–10919. https://doi.org/10.1073/pnas.1505655112
Radman, M., Wagner, R., Glickman, B., & Meselson, M. (1980). DNA methylation, mismatch correction and genetic stability. In: Progress in environmental mutagenesis (pp. 121–130).
Reenan, R., & Kolodner, R. D. (1992). Isolation and characterization of two Saccharomyces cerevisiae genes encoding homologs of the bacterial HexA and MutS mismatch repair proteins. Genetics, 132(4), 963–973. https://doi.org/10.1093/genetics/132.4.963
Reyes, G. X., Zhao, B., Schmidt, T. T., Gries, K., Kloor, M., & Hombauer, H. (2020). Identification of MLH2/hPMS1 dominant mutations that prevent DNA mismatch repair function. Communications Biology, 3(1), 1–14. https://doi.org/10.1038/s42003-020-01481-4
Robertson, A., Pattishall, S. R., & Matson, S. W. (2006). The DNA binding activity of MutL is required for methyl-directed mismatch repair in Escherichia coli. Journal of Biological Chemistry, 281(13), 8399–8408. https://doi.org/10.1074/jbc.M509184200
Sacho, E. J., Kadyrov, F. A., Modrich, P., Kunkel, T. A., & Erie, D. A. (2008). Direct visualization of asymmetric adenine nucleotide-induced conformational changes in MutLα. Molecular Cell, 29(1), 112–121. https://doi.org/10.1016/j.molcel.2007.10.030
Schmutte, C., Marinescu, R. C., Sadoff, M. M., Guerrette, S., Overhauser, J., & Fishel, R. (1998). Human exonuclease I interacts with the mismatch repair protein hMSH2. Cancer Research, 58(20), 4537–4542.
Schmutte, C., Sadoff, M. M., Shim, K. S., Acharya, S., & Fishel, R. (2001). The interaction of DNA mismatch repair proteins with human exonuclease I. The Journal of Biological Chemistry, 276(35), 33011–33018. https://doi.org/10.1074/jbc.M102670200
Smith, C. E., Mendillo, M. L., Bowen, N., Hombauer, H., Campbell, C. S., Desai, A., Putnam, C. D., & Kolodner, R. D. (2013). Dominant mutations in S. cerevisiae PMS1 identify the Mlh1-Pms1 endonuclease active site and an exonuclease 1-independent mismatch repair pathway. PLoS Genetics, 9(10), e1003869. https://doi.org/10.1371/journal.pgen.1003869
Su, S. S., & Modrich, P. (1986). Escherichia coli mutS-encoded protein binds to mismatched DNA base pairs. Proceedings of the National Academy of Sciences of the United States of America, 83(14), 5057–5061. https://doi.org/10.1073/pnas.83.14.5057
Tishkoff, D. X., Amin, N. S., Viars, C. S., Arden, K. C., & Kolodner, R. D. (1998). Identification of a human gene encoding a homologue of Saccharomyces cerevisiae EXO1, an exonuclease implicated in mismatch repair and recombination. Cancer Research, 58(22), 5027–5031.
Umar, A., Buermeyer, A. B., Simon, J. A., Thomas, D. C., Clark, A. B., Liskay, R. M., & Kunkel, T. A. (1996). Requirement for PCNA in DNA mismatch repair at a step preceding DNA resynthesis. Cell, 87(1), 65–73. https://doi.org/10.1016/s0092-8674(00)81323-9
Viswanathan, M., Burdett, V., Baitinger, C., Modrich, P., & Lovett, S. T. (2001). Redundant exonuclease involvement in Escherichia coli methyl-directed mismatch repair. The Journal of Biological Chemistry, 276(33), 31053–31058. https://doi.org/10.1074/jbc.M105481200
Welsh, K. M., Lu, A. L., Clark, S., & Modrich, P. (1987). Isolation and characterization of the Escherichia coli mutH gene product. The Journal of Biological Chemistry, 262(32), 15624–15629. https://doi.org/10.1016/s0021-9258(18)47772-1
Wilson, T. M., Vaisman, A., Martomo, S. A., Sullivan, P., Lan, L., Hanaoka, F., Yasui, A., Woodgate, R., & Gearhart, P. J. (2005). MSH2-MSH6 stimulates DNA polymerase eta, suggesting a role for A: T mutations in antibody genes. The Journal of Experimental Medicine, 201(4), 637–645. https://doi.org/10.1084/jem.20042066
Wu, H., Zeng, H., Lam, R., Tempel, W., Kerr, I. D., & Min, J. (2015). Structure of the human MLH1 N-terminus: implications for predisposition to Lynch syndrome. Acta Crystallographica. Section f, Structural Biology Communications, 71(8), 981–985. https://doi.org/10.1107/S2053230X15010183
Yang, X. W., Han, X. P., Han, C., London, J., Fishel, R., & Liu, J. (2022). MutS functions as a clamp loader by positioning MutL on the DNA during mismatch repair. Nature Communications, 13(1), 1–17. https://doi.org/10.1038/s41467-022-33479-3
Yang, X. W., & Liu, J. (2021). Observing protein one-dimensional sliding: methodology and biological significance. Biomolecules, 11(11), 1618. https://doi.org/10.3390/biom11111618
Yoshioka, K., Yoshioka, Y., & Hsieh, P. (2006). ATR kinase activation mediated by MutSα and MutLα in response to cytotoxic O6-methylguanine adducts. Molecular Cell, 22(4), 501–510. https://doi.org/10.1016/j.molcel.2006.04.023
Zhang, Y., Yuan, F., Presnell, S. R., Tian, K., Gao, Y., Tomkinson, A. E., Gu, L., & Li, G. M. (2005). Reconstitution of 5’-directed human mismatch repair in a purified system. Cell, 122(5), 693–705. https://doi.org/10.1016/j.cell.2005.06.027
Acknowledgements
This work was supported by the National Key R&D Program of China (2021YFA1300503), the Chinese Academy of Sciences (CAS) (YSBR-009), the National Natural Science Foundation of China (32071283), the Shanghai Municipal Commission for Science and Technology (20JC1410300), the Natural Science Foundation of Shanghai (20ZR1474100) and the Shanghai Pujiang Program (20PJ1414500) to J. Liu.
Author information
Authors and Affiliations
State Key Laboratory of Molecular Biology, Shanghai Key Laboratory of Molecular Andrology, CAS Center for Excellence in Molecular Cell Science, Shanghai Institute of Biochemistry and Cell Biology, University of Chinese Academy of Sciences, Chinese Academy of Sciences, 320 Yueyang Road, Shanghai, 200031, China
Xiao-Peng Han, Xiao-Wen Yang & Jiaquan Liu
Contributions
Conceptualization: X-PH and JL; investigation (literature review): X-PH; writing—original draft preparation: X-PH; writing—review and editing: X-PH, X-WY and JL; figure preparation: X-PH; supervision, JL; All authors have read and agreed to the published version of the manuscript.
Corresponding author
Correspondence to Jiaquan Liu.
Ethics declarations
Conflict of interest
The authors declare no competing interests.
Rights and permissions
Springer Nature or its licensor (e.g. a society or other partner) holds exclusive rights to this article under a publishing agreement with the author(s) or other rightsholder(s); author self-archiving of the accepted manuscript version of this article is solely governed by the terms of such publishing agreement and applicable law.
About this article
Cite this article
Han, XP., Yang, XW. & Liu, J. MutS and MutL sliding clamps in DNA mismatch repair. GENOME INSTAB. DIS. (2022). https://doi.org/10.1007/s42764-022-00094-x
Received19 October 2022
Revised15 November 2022
Accepted21 November 2022
Published09 December 2022
DOIhttps://doi.org/10.1007/s42764-022-00094-x
Share this article
Anyone you share the following link with will be able to read this content:
Get shareable linkKeywords
DNA mismatch repair
MutS sliding clamp
MutL sliding clamp
MSH
MLH/PMS
用户登录
还没有账号?
立即注册