Telomere-based treatment strategy of cardiovascular diseases: imagination comes to reality
Review Article
Published: 16 February 2024
Mohammed Abdel-Gabbar & Mohamed G. M. Kordy Genome Instability & Disease Vol5 Issue 2
Abstract
The significance of telomere/telomerase biology in the pathogenesis of age-related cardiovascular diseases (CVDs), such as atherosclerosis, hypertension, myocardial infarction (MI), and heart failure, has been increasingly highlighted in recent years. The activation of the DNA damage response (DDR) due to the presence of short telomeres is believed to be a significant upstream signal responsible for inducing a permanent cessation of the cell cycle in cardiomyocytes. Heart failure (HF) is a condition that arises due to the restricted regenerative capacity of the elderly and injured mammalian heart. This limitation may be related to the decreased proliferative potential of cardiac stem cells (CSCs) and cardiomyocytes. The association between CVDs and shorter telomeres provides a foundation for developing therapeutic techniques aimed at elongating telomeres and subsequently restoring the proliferative ability of the adult mammalian heart. This phenomenon offers intriguing prospects for the treatment and prevention of cardiovascular disease (CVD). Further investigation into telomerase gene therapy in the field of cardiac regenerative medicine is justified based on the encouraging outcomes shown in mice models, whereby the reactivation of telomerase in the heart after MI has demonstrated beneficial effects.
Telomere in nature
The basic repetitive sequence (TTAGGG in humans) that makes up telomeres, repetitive structures in DNA linked to proteins at the ends of eukaryotic chromosomes, controls cellular replication and proliferation, prevents genetic data loss, and keeps chromosomes from uniting forces during mitosis. Comparable to the points on shoelace ends that keep them from coming undone are telomeres (Fig. 1) (Kaur et al., 2016). In 1971, Alexey Olovnikov referred to telomeres as "the Achilles' heel" of DNA due to their possible impact on aging (Olovnikov, 1973). The current range of human telomere sequences is 5000–15,000 bp (Chilton et al., 2017). A person's biological age is measured by telomere length (TL), a "mitotic clock" that differs from their chronological age. Specifically, the inherent potential of TL lies in its ability to forecast the onset of disease (Meyer et al., 2018).
Fig. 1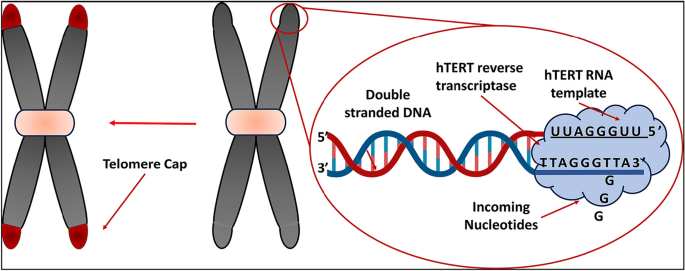
Telomeres can be viewed as the tips on the ends of shoelaces
Shelterin multiprotein complex
A telomeric loop, or t-loop, is a structure that forms when the 3' G-strand overhang folds back and invades a duplex region. This binds the overhang in place (Blackburn, 2001; Griffith et al., 1999; Liu et al., 2004). As shown in Figs. 2 and 3, a group of telomere-specific proteins combine to form the telosome, also called the shelterin multiprotein complex, a six-protein complex that maintains the shape of the telomere end loop (Lange, 2005; Liu et al., 2004). Proteins that directly bind to single-stranded TTAGGG, like POT1, or to double-stranded telomeric repeats, such the telomere binding factors TRF1 and TRF2, make up shelterin. Three more proteins—TIN2, TPP1, and RAP1—connect these proteins. Where TPP1 is TIN2 and POT1 interacting Protein. The shelterin complex is responsible for controlling the overall protective function of telomeres, also referred to as the "capping function" (Lange, 2002; Sandell & Zakian, 1993). The role of this mechanism encompasses the protection of chromosomal termini against enzymatic degradation, the prevention of fusion between chromosomal ends, and the avoidance of recognition of chromosomal DNA as double-stranded breaks, hence averting an erroneous activation of the DNA damage response (DDR). Furthermore, it has been postulated that telomeres undergo a transition from a "capped" to a "uncapped" state during the process of cell division (Blackburn, 2000). Cell division and oxidation are the two main mechanisms that affect TL. It is estimated that around 50 base pairs are lost during cell division due to the end-replication problem (Proctor & Kirkwood, 2002).
Fig. 2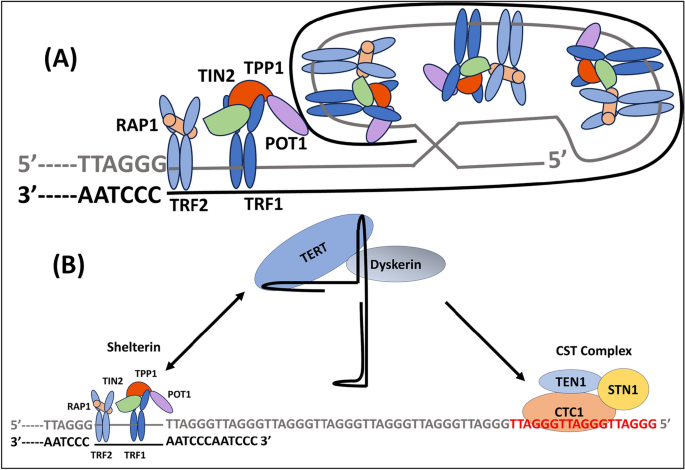
Telomere structure and lengthening. A The human telomeric DNA contains the TTAGGG hexanucleotide in several kilobases, or up to 15 kb. The double-stranded telomeric DNA's 5ʹ–3ʹ G-rich strand (light grey) and matching 3ʹ–5 C-rich strand (dark grey) are shown. When the G-strand 3' overhang at the end of telomeres invades the double-stranded telomeric sequence, a closed structure known as a telomeric-loop (t-loop) is created. The telomere body is surrounded by shelterin complexes composed of the subunits TRF1, TRF2, RAP1, TIN2, TPP1, and POT1. POT1 binds to single-stranded telomeric DNA, while TRF1 and TRF2 directly bind to double-stranded telomeric DNA. B At the telomere end, the shelterin complex's TPP1 component serves as a bridge factor between telomeres and telomerase. At the conclusion of every cell cycle, telomerase inserts several telomeric repeats at the 3ʹ overhang (-TTAGGG- in red). When newly produced repetitions are bound, the complex CTC1-STN1-TEN1 (CST) suppresses telomerase activity
Fig. 3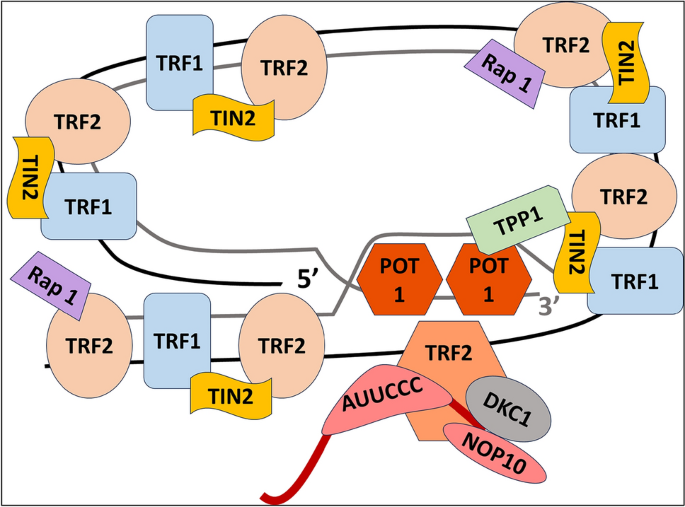
Multiprotein complex shelterin
Because the replication mechanism cannot duplicate DNA's extreme ends, TL reduces with time. The "end-replication problem" is the term used to describe this (Blackburn, 2000). The phenomenon of telomere shortening in response to oxidative stress is an unexpected finding (Zglinicki et al., 2000). In the absence of a reparative process, DNA sequences would progressively diminish with each replicative phase until reaching a certain threshold, triggering the cessation of cell division. The occurrence of a single-stranded break at different locations may result in varying effects on TL, ranging from a loss of up to 1000 base pairs of telomeric DNA to no influence at all (Proctor & Kirkwood, 2002). Since TL varies significantly across species, findings from animal models could not necessarily translate to human situations (Sanders & Newman, 2013).
European, Russian, and US citizens telomeres
The mean leucocyte telomere length (LTL) of eleven European countries varied significantly, according to the European Atherosclerosis Research Study II (Eisenberg et al., 2011). across 5.1 kb (Italy) to 18.6 kb (Belgium), there was a significant variation in TL, which made ordering comparisons across populations very impossible. LTL was assessed in 608 patients with coronary artery disease in the Heart and Soul experiment (Farzaneh-Far et al., 2010a) at baseline and 5 years thereafter. The mean LTL dropped to 5.3 kb after five years from 5.5 kb at baseline; however, only half of the patients showed LTL shortening.
Several studies have explored the relationship between LTL and various health outcomes, including mortality and CVD (Xiong et al., 2023). One study conducted in a Russian population found that individuals with longer LTL had a reduced risk of all-cause mortality and death from coronary heart disease (CHD) (Stefler et al., 2018). Similar findings were observed in a nationally representative sample of individuals in the United States, where LTL was linearly associated with CVD risk. Additionally, this study found that the relationship between LTL and CVD risk was influenced by factors such as education and hypertension. Another study focused specifically on individuals with metabolic syndrome (MetS) in the US and found that shorter TL was associated with a higher risk of all-cause and CVD mortality (Xu et al., 2020). These findings suggest that LTL may serve as a measure of or a contributor to human health in different populations, and further research is needed to understand the underlying mechanisms and clinical implications of these associations.
The naturally occurring enzyme telomerase aids in telomere repair. With the exception of blood mononuclear cells in children (Engelhardt et al., 1998), certain cardiovascular system cells, stem cells, germ cells, and hair follicles (Zurek et al., 2016), it is expressed in relatively few somatic tissues. Conversely, tumour cells reactivate telomerase expression to evade replicative senescence and attain immortality (Martínez & Blasco, 2011).
Reverse transcriptase (TERT) and its primer, the telomerase RNA component (TERC), make up the ribonucleoprotein telomerase, which functions to add bases to the ends of telomeres. Because of their telomerase activity, these cells seem to be eternal. Somatic cells experience replicative senescence when they reach the Hayflick limit (Hayflick & Moorhead, 1961), which is a number of divisions that is particular to a donor. Ectopic telomerase expression may surpass this limit, which results in considerable telomere shortening (Bodnar et al., 1979).
The presence of oxidized nucleic acids, proteins, and lipids accelerates aging, according to the free radical theory of ageing (McCully, 2018). The neuroendocrine theory of aging, on the other hand, attributes these alterations to the hypothalamus's diminished feedback control of hormone production (McCully, 2018). It is worth mentioning that telomerase depletion with age is connected to a decrease in nitric oxide production by nitric oxide synthase (McCully, 2018).
Because TL decreases as oxidative stress increases (), reducing oxidative stress slows telomere shortening and delays replicative senescence (Passos et al., 2007). Oxidation has a major impact on telomeric DNA owing to a particular deficiency in base excision repair (Petersen et al., 1998; Zglinicki et al., 2000).
Telomeres of insufficient length have been shown to be associated with the development of lung fibrosis, nail dystrophy, and leukoplakia. It is important to note that these conditions are distinct from the natural aging process (Sanders & Newman, 2013). It is important to acknowledge that cellular aging may also occur as a result of telomere-independent signaling (Sanders & Newman, 2013).
The current inquiry pertains to the potential causal relationship between shortened telomeres and the subsequent release of inflammatory proteins that facilitate the process of aging. This may provide insight into other variables beyond cellular senescence and oxidative damage.
Leukocytes
The well-known systemic illness known as atherosclerosis is characterized by low-grade systemic oxidative stress and chronic inflammation that develops with aging. Different kinds of leukocytes are essential to this physiological process. There was no text provided by the user to alter. Blood leukocytes may be divided into two primary categories: myeloid and lymphoid. Natural killer (NK) cells, B lymphocytes, and CD4 and CD8 T lymphocytes (T cells) are all descended from lymphoid progenitor cells. Nevertheless, granulocytes (neutrophils, basophils, and eosinophils), monocytes, macrophages, and mast cells are all developed by myeloid progenitor cells in contrast (Hoffmann & Spyridopoulos, 2011).
Hematopoietic stem cells (HSCs) constitute a minute fraction, around 0.01%, of the total population of cells present in the bone marrow. The uninterrupted operation of HSCs, sustained by their inherent capacity for self-renewal (Lansdorp, 1998), is essential for the ongoing production of billions of fresh blood cells on a daily basis (about 200 billion cells per day in adults). It has been shown that granulocytes, except for neutrophils found in coronary plaques, do not exhibit telomerase activity (Narducci et al., 2007). The rate at which lymphocytes' TL decreases is significantly swifter when contrasted with the decline in granulocytes' TL (53 base pairs per year against 39 base pairs per year) (Hoffmann et al., 2009; Rufer et al., 1999). The study revealed that females had significantly longer telomeres in the lymphocyte population as compared to males (Hoffmann et al., 2009). Guan et al. (Guan et al., 2007) observed a more rapid decline in TL in males compared to females within the Japanese population, up to the age of 50. The reversal of this ratio was seen in postmenopausal women.
In 2001, Samani et al., (2001) made the discovery that individuals diagnosed with coronary heart disease (CHD), a prevalent age-related condition, had reduced leukocyte TL compared to a group of healthy individuals serving as controls. In 2003, Cawthon et al. conducted a study that established the validity of the association between shorter LTL and elevated death rates attributed to CVD and infections (Cawthon et al., 2003). Subsequently, scholars have endeavored to ascertain the use of LTL as a biomarker in prognosticating forthcoming cardiac events, notwithstanding the presence of methodological challenges in replicating divergent LTL findings across various research facilities. Individuals with a shorter TL were shown to have a significantly increased likelihood of developing coronary artery disease, with almost double the risk compared to those with longer telomeres. The use of pravastatin medicine has the potential to completely mitigate this particular danger (Brouilette et al., 2007).
The measurement of LTL serves as a biomarker for the combined impact of inflammation and oxidative stress experienced throughout adulthood. Research has shown associations between LTL and several health conditions such as atherosclerosis, stroke, and accelerated aging (Jin et al., 2018). LTL is a significant contributor to the process of vascular aging, whereas those with shorter LTL have a diminished capacity for repair. The observed disparity in TL between muscle tissue and leukocytes in adult individuals, which amounts to around 1.5 kilobase pairs (Kbp), may be attributed to the attrition of TL throughout the growth period, namely during childhood and adolescence. Individuals suffering from chronic pain may have reduced TL compared to individuals of the same age without such discomfort. A significant association was seen between reduced TL in leukocytes and the presence of CVD, as well as risk factors including elevated pulse pressure, accelerated vascular aging, obesity, vascular dementia, diabetes, and coronary artery disease (Epel et al., 2009).
Epigenetic age acceleration refers to the discrepancy seen between the epigenetic clock and chronological age. This phenomenon has been associated with mortality, regardless of an individual's chronological age (Perna et al., 2016; Smith et al., 2019). On other means, epigenetic age acceleration—DNA methylation-based biological aging—is linked to cardiovascular mortality. Little is known about its association to hypertensive heart, kidney, brain, and peripheral artery disease. Several research have examined the association between shorter LTL and death rates. While some studies (Bodnar et al., 1996; Hooijberg et al., 2000; Iancu et al., 2008; Rufer et al., 2001; Weng et al., 1996) have shown a positive correlation between shorter LTL and greater mortality rates, other studies (Dagarag et al., 2003; Haendeler et al., 2004; Lange et al., 1990; Valenzuela & Effros, 2002; Zglinicki, 2002) have not seen such a relationship. Epel et al. (2009) made a significant observation that a shorter length of telomeres, known as LTL, exhibited a correlation with cardiovascular disease-specific mortality in women, but no such association was seen in males. Furthermore, the study did not find any relationship between LTL and overall mortality. In summary, it may be inferred that low testosterone levels exhibit a moderate association with the overall likelihood of mortality and perhaps a stronger correlation with death specifically attributed to cardiovascular or infectious diseases (Hoffmann & Spyridopoulos, 2011).
In accordance with Jiang et al. (Jiang et al., 2022), the robust predictability of mortality persists in individuals afflicted with CVD, even when accounting for epigenetic age acceleration. The associations with mortality were found to be mediated by Angiopoietin-2, a regulator of endothelial permeability and angiogenic functions. This implies that specific vascular pathophysiology could serve as a link between accelerated epigenetic aging and heightened mortality risks.
Smith et al. (2019) observed a link between epigenetic age acceleration and kidney, heart, and peripheral artery damage. Urinary albumin-creatinine ratio, heart wall thickness, and ankle-brachial index were linked with intrinsic epigenetic aging. External epigenetic aging markers were linked to left ventricular mass index. Most of these relationships were reduced after controlling for blood pressure, body mass index (BMI), diabetes, and smoking. The therapeutic efficacy of epigenetic age acceleration in quantifying target organ damage across organ systems needs more study. Knowing that epigenetic mechanisms like DNA methylation, histone acetylation, and microRNA regulate gene expression, which changes with age (Peters et al., 2015). DNA methylation, specifically cytosine-5 methylation of CpG dinucelotides, has been used to determine epigenetic age acceleration, the difference between biological and chronological age (Hannum et al., 2013; Horvath, 2013).
In López-Armas et al.‘s study on multiple sclerosis (MS) (López-Armas et al., 2023) showed that LTL is good biomarker of disability in relapsing–remitting MS (RRMS) and is correlated with mitochondrial DNA copy number (mtDNA-CN) in mild-moderate RRMS patients. They included 10 healthy controls, 75 RRMS patients, 50 with mild to moderate disabilities on the Expanded Disability Status Scale (EDSS) (0–3), and 25 with severe disabilities (3.5–7). To measure absolute LTL and absolute mtDNA-CN, they used the Real-Time Polymerase Chain Reaction (qPCR) method.
The effect of free radicals on telomere length
There are three reasons why it is seen that telomeres develop oxidative single-strand DNA damage at a faster rate compared to the remainder of the genome. Guanine triplet-containing DNA sequences, similar to telomere repeat sequences, have a high susceptibility to oxidative modification (Oikawa et al., 2001; Saretzki et al., 2003; Zglinicki et al., 2000). Furthermore, the efficiency of repairing single-strand DNA breaks in telomeres is much lower compared to the rest of the genome (Petersen et al., 1998). Oxidative stress has been shown to impede the maintenance of telomeres in some cell types via the inhibition of telomerase (Jakob et al., 2008).
Based on the findings of reference (O’Donovan et al., 2011a), a significant association has been observed between shortened LTL and the occurrence of systemic inflammatory response. Elevated concentrations of interleukin-6 (IL-6) or tumor necrosis factor-alpha (TNF-α), or both, have been associated with a heightened susceptibility to shortened LTL. Nevertheless, a correlation between C-reactive protein and the aforementioned relationship was not found (Njajou et al., 2009). In general, these results might be seen as more evidence supporting the association between chronic, mild inflammation, shortening of TL, and the emergence of disorders connected to inflammation, such as coronary heart disease (Hoffmann & Spyridopoulos, 2011).
The telomeres of endothelial cells derived from atherosclerotic plaques were shown to be shorter compared to those obtained from non-atherosclerotic sites within the same individual (Matthews et al., 2006; Ogami et al., 2004). The length of telomeres LTL was shown to be significantly reduced in persons who had an MI before the age of 50, compared to a control group of healthy individuals. There is a correlation between shorter leukocyte telomeres and an elevated risk of MI (Shammas, 2011). According to reference (Brouilette et al., 2007), the administration of pravastatin medicine has the potential to completely mitigate this risk. Moreover, it has been shown that TL is reduced in persons diagnosed with congestive HF (Harst et al., 2007).
There are other age-related changes that are not only associated with LTL. There is some evidence to suggest that LTL may have a weak or negligible association with pulmonary function (Harris et al., 2006; Mather et al., 2010) and bone mineral density (Sanders et al., 2009; Tang et al., 2010; Valdes et al., 2007), which are markers of tissue decline and structural degradation that occur with aging, regardless of any underlying disease. In addition, it should be noted that there is currently no established correlation between grip strength, a metric used to measure physical function, and LTL as shown by previous studies (Bekaert et al., 2005; Harris et al., 2006; Mather et al., 2010).
Aging
The process of ageing is multifaceted and leads to progressive decline in cellular, tissue, and organismal functions, ultimately leading in reduced physical fitness and heightened susceptibility to death. In addition to observable manifestations of the aging process, such as graying hair, wrinkled skin, muscle loss, and increasing body fat, the vulnerability to diseases increases with advancing age in the human population (Martínez & Blasco, 2018). Several molecular mechanisms of aging have been found, including telomere shortening, cellular senescence, genomic instability, stem cell exhaustion, mitochondrial failure, epigenetic modifications, unregulated nutrition sensing, changed cellular communication, and loss of proteolysis (López-Otín et al., 2013).
The primary factor believed to contribute to the process of aging is the presence of shortened telomeres. This is due to their ability to initiate a continuous DDR, which subsequently leads to the cessation of growth or programmed cell death, also known as apoptosis (Sarin et al., 2005). The significance of replicative senescence resulting from short telomeres has been well acknowledged (Kovalenko et al., 2010; Park, et al., 2009). The concept of replicative senescence has been proposed as an evolutionary mechanism that evolved to provide protection against cancer, while simultaneously contributing to the development of age-related diseases (Jakob et al., 2008). The accumulation of senescent cells over time leads to an elevated production of proinflammatory cytokines, proteases, and insoluble extracellular matrix components (Haendeler et al., 2004). The substances released by senescent cells have a paracrine effect on adjacent cells, promoting degenerative and hyperproliferative changes that disrupt the local tissue environment and lead to the development of chronic inflammation (Haendeler et al., 2004). The process of telomere shortening has been seen to result in stem cell dysfunction, leading to a diminished capacity for self-renewal (Blasco et al., 1997; Collado et al., 2007; Flores et al., 1979, 2008; Harley et al., 1990; Lindsey et al., 1991; Liu et al., 2007; Marion et al., 2009; Schaetzlein et al., 2004). The impairment of mitochondrial biogenesis and function has been found to be associated with telomere dysfunction through the activation of p53, which suppresses the expression of PGC1-alpha and PGC1-beta (peroxisome proliferator-activated receptor gamma coactivators 1-alpha and 1-beta) (Sahin et al., 2011). The process of telomere shortening has been seen to result in epigenetic modifications inside the chromatin regions of telomeres and subtelomeres (Benetti et al., 2007). The depletion of heterochromatin, specifically, may lead to a decline in cellular differentiation and identity, hence increasing the risk of cancer and compromising the process of pluripotency induction (Marion et al., 2009; Vera et al., 2008). The length of telomeres may also impact human health by regulating gene expression via telomere position effects, which include the spreading of telomeric heterochromatin to silence adjacent genes (Robin et al., 2014; Stadler et al., 2013).
The presence of shortened telomeres in animals without telomerase enzyme activity is associated with a reduction in the size of pancreatic islets. This reduction subsequently impairs the generation of insulin and contributes to the development of diabetes mellitus (Kuhlow et al., 2010). Numerous studies have provided data supporting the association between telomerase overexpression and elevated levels of fasting insulin as well as enhanced glucose absorption (Bernardes de Jesus et al., 2012). In the context of addressing the indications of aging, it is plausible that intervention at the telomere level alone might potentially be effective in achieving reversal. The first indication that shorter telomeres contribute to age-related diseases and influence lifespan was derived from research conducted on telomerase-deficient animals. These mice had premature aging characteristics in many highly proliferating compartments such as the heart and brain (Blasco et al., 1997; Ferrón et al., 2004; Herrera et al., 1999; Lee et al., 1998; Leri et al., 2003). The potential use of adeno-associated vectors (AAV) as a therapeutic modality for prolonging lifespan and delaying age-related ailments relies on a methodology that induces telomerase activation in mature tissues (Bär et al., 2014; Bernardes de Jesus & Blasco, 2013).
Accelerated aging in certain rare genetic diseases has been attributed to several factors, including alterations in DNA repair mechanisms, depletion of the coenzyme NAD+ , and disrupted metabolism of methionine and homocysteine (McCully, 2018). The current hypothesis proposes that the abnormalities seen in cellular ageing and carcinogenesis, namely in oxidative phosphorylation and mitochondrial dysfunction, may be attributed to the loss of the thioretinaco ozonide oxygen ATP complex resulting from the opening of the mitochondrial permeability transition pore (mPTP). When endothelial cells that have been grown in a controlled environment are subjected to homocysteine, they experience cellular senescence, which is characterized by telomere shortening and an elevation in the levels of acidic -galactosidase, a well-known indicator of cellular senescence (McCully, 2018).
Senescence, fibrosis, inflammation, and stem cell depletion result from telomere shortening in the context of a functioning p53 checkpoint. Aging and degenerative and inflammatory illnesses result from these mechanisms. Age-related disorders may be prevented by telomerase activators and senolytics. Short telomeres cause telomeric fusion and break-fusion-bridge cycles. With no p53 checkpoint, these events cause carcinogenesis. Further telomerase activation causes invasion and metastases. Telomerase inhibitors and senolytics stop tumor growth, invasion, and metastasis (Chakravarti et al., 2021). Wong et al. also (2014) suggested that increased systemic inflammation, consistent with vascular injury, is associated with decreased LTL. Hence, it is crucial to acknowledge that examining the epigenetic age of blood confirms the health benefits associated with a diet rich in plants and lean meats, moderate alcohol consumption, regular physical exercise, and education (Quach et al., 2018; Zhang et al., 2023). Conversely, it also underscores the health risks linked to obesity and metabolic syndrome.
Immune system aging may be vulnerable to environmental and lifestyle differences, and the many connections discovered with Extrinsic epigenetic age acceleration (EEAA) point to a tight relationship with traditional ideas of metabolic health according to a group of researchers in the USA (Quach et al., 2018). The sparse association data for intrinsic epigenetic age acceleration (IEAA), on the other hand, lend credence to the idea that cell-intrinsic aging is mostly dictated by genetically controlled developmental processes or intrinsic aging, and that it stays relatively constant across time. We also discovered that changes in both EEAA and IEAA are strongly linked to changes in BMI using longitudinal data from the WHI. This indicates that both forms of epigenetic aging may react to changes in lifestyle, specifically changes in obesity. Overall, their findings corroborate the preventive effects of consuming fish, poultry, and alcohol, as well as the risks of obesity and dyslipidemia, and the benefits of exercising and receiving an education.
The impact of non-genetic factors on telomere length
Several investigations carried out in the last several years have shown that non-genetic factors, including psychological, environmental, and behavioral variables, may influence the maintenance of TL homeostasis, therefore affecting both the duration of a healthy lifespan and the occurrence of diseases (Blackburn et al., 1979; Starkweather et al., 2014). Therefore, it has been shown that certain lifestyle factors such as smoking, an unhealthy diet characterized by high cholesterol and alcohol intake, as well as obesity, may lead to the shortening of telomeres via the induction of tissue inflammation and oxidative stress (Révész et al., 2015; Strandberg et al., 2011a, 2011b; Valdes et al., 2005; Verde et al., 2015). Research has shown that stress may accelerate the process of telomere shortening in leukocytes (Entringer et al., 2011; Epel et al., 2004; O’Donovan et al., 2011b; Price et al., 2013). Individuals who are classified as obese have shorter telomere lengths (TLs) in comparison to individuals who are classified as lean. Likewise, obesity is a significant risk factor for the development of chronic ailments, including cardiovascular and neurological problems (Ashrafian et al., 2013; Svačina, 2020).
In contrast, a number of dietary practices, meditation techniques, adequate sleep patterns, and regular physical exercise have been scientifically shown to mitigate the destruction of telomeres and decelerate the process of aging (Farzaneh-Far et al., 2010b; Werner et al., 2009).
The findings suggest that engaging in exercise training may have a protective effect on cardiac telomeres, as shown by previous studies (Ludlow et al., 2012; Waring et al., 2014). This implies that TL and the genes associated with telomeres play a significant role in the adaptability of the heart (Booth & Charchar, 2017). However, the observed augmentation in cardiac mass and relative heart weight during prolonged exercise is attributed to cardiomyocyte hypertrophy rather than hyperplasia (Booth et al., 2018). In contrast, physical exercise has the potential to serve as a therapeutic approach prior to the attainment of a certain length of telomeres, beyond which cell loss becomes permanent (Booth et al., 2018).
There is a documented association between race/ethnicity and TL, whereby individuals of Hispanic and black descent have longer telomeres (Lynch et al., 2016). There is a significant divergence of opinion on the extent to which TL inheritance is mostly linked to either paternal or maternal inheritance patterns (Eisenberg, 2014). Meta-analyses have shown that females possess longer telomeres compared to men, as evidenced by the examination of the association between sex and TL (Canela et al., 2007; Gardner et al., 2014).
CVDs and telomeres
Telomere syndromes, also known as telomeropathies, arise as a consequence of genetic abnormalities that disrupt the mechanisms responsible for telomere maintenance and repair (Booth & Charchar, 2017). Inherited telomeropathies exhibit discernible patterns that may include several manifestations, including but not limited to compromised immunological function resulting from depletion of bone marrow stem cell pool, particular malignancies, lung fibrosis, gastrointestinal complications, liver cirrhosis, and neuropsychiatric disorders (Farzaneh-Far et al., 2010a).
Telomeres, repeated nucleoprotein structures at chromosome ends, are critical for heart function. The greatest cause of mortality globally is heart disease, which is caused by cellular senescence and apoptosis triggered by a reduction in TL. Research on TL has shown dramatic changes in cardiac TL throughout the beginning and development of heart disease. The loss of telomeric proteins, oxidative stress, and hypoxia all contribute to a reduction in cardiac TL and cardiac performance (Booth & Charchar, 2017). However, the loss of cardiac telomeres and the advancement of heart disease may be halted with the use of antioxidants, calorie restriction, and exercise. Transforming growth factor β in the heart suggests proliferative capacity and may contribute to cardiac rejuvenation. However, concerns concerning the reliability and potential for bias in animal models must be taken into account. It is hoped that by better understanding telomeric pathways in heart development, cardiac dysfunction may be avoided and regeneration after damage can be accelerated.
CVD encompasses a range of heart-related disorders, including MI, atherosclerosis, hypertension, and heart failure. CVDs are the leading cause of death and the most significant contributor to chronic disability on a global scale (Benjamin et al., 2017). According to the cited study (Mwasongwe et al., 2017), there is a significant correlation between the reduction in TL, measured in shorter kilobase pairs, in blood cells and an increased risk of MI and stroke. Specifically, each shortened kilobase pair of telomere is linked with a threefold higher chance of experiencing these cardiovascular events. There is evidence suggesting an association between reduced TL in leukocytes and the occurrence of stroke, MI, as well as type 2 diabetes mellitus (D’Mello et al., 2015). The relationship between TL and atherosclerotic vascular disease has been seen in population research (Butt et al., 2010; D’Mello et al., 2015).
The postulated explanation for the majority of cardiac telomere attrition across an individual's lifespan is oxidative stress, while the heart has a low mitotic index (Hemmeryckx et al., 2016; Leri et al., 2003). It is important to acknowledge that changes in TL need a significant amount of time to become apparent. Several studies have shown a decline in cardiac TL at a rate of 20 base pairs per year (Terai et al., 2013). However, contrasting findings have indicated that TL remains unchanged with age in the hearts of healthy individuals (Sharifi-Sanjani et al., 2017). In contrast, previous studies have shown a significant correlation between TL in isolated cardiomyocytes and TL in the whole of cardiac tissue (Marques et al., 2016). Nevertheless, there exists considerable variability among individuals in terms of TL.
Studies examining TL in biopsied cardiac tissues and cardiomyocytes have shown a clear association between reduced TL in the adult heart and various cardiac diseases, such as HF (Booth & Charchar, 2017; Sharifi-Sanjani et al., 2017; Terai et al., 2013). The phenomenon of telomere shortening was shown to be exclusive to cardiomyocytes, as opposed to cardiac smooth muscle cells within the same cardiac organ (Sharifi-Sanjani et al., 2017). Indeed, it has been shown that cardiomyocyte precursor cells with elongated telomeres have the capacity to generate daughter cells that are both functional and exhibit an extended lifespan (Kajstura et al., 2010). The involvement of cardiomyocytes in heart disease raises the question of whether the presence of shorter telomeres is a consequence of the injury-induced proliferation of these cells or whether the presence of short telomeres really contributes to heart damage. Both ideas are deemed reasonable.
Research on human vascular studies has shown that persons with atherosclerosis had shorter telomeres in their coronary endothelium cells compared to age-matched healthy controls. This finding suggests that the shortening of endothelial telomeres may contribute to the development of atherosclerosis (Ogami et al., 2004). Nevertheless, research conducted on human abdominal aortic endothelial cells, with a focus on age and atherosclerosis, revealed that there was no significant association between TL and the severity of atherosclerosis. Instead, the correlation was shown to be more closely related to cellular turnover (Okuda et al., 2000).
Telomeres are ribonucleoprotein structures that protect chromosomes and genetic information. They shorten with mitotic cell division, but telomerase elongates them in specific cells. Mutations in telomere maintenance and function differ between humans and mice. Mice have longer telomeres but shorter lifespans, while humans have more organ regeneration defects and cancer development (Zaragoza et al., 2011). Telomerase deficiency accelerates telomere shortening, but its effects vary due to TL differences.
There is growing recognition that dyskeratosis congenita, a telomere condition caused by germline mutations in telomere biology genes, manifests as several cardiovascular illnesses including cardiac fibrosis, dilated cardiomyopathy, MI, and pulmonary arteriovenous malformations (Khincha et al., 2017). Genetic data obtained using Mendelian randomization approaches establishes a connection between several genetic variations found in telomere repairs genes (TERT, TERC, NAF1, STN1, and RTEL1) and an elevated susceptibility to heart disease (Codd et al., 2013; Scheller Madrid et al., 2016). The occurrence of insulin resistance is more prevalent among individuals with shorter telomeres, as shown by previous studies (Verhulst et al., 2016; Weischer et al., 2014).
Cardiac regeneration
The heart, being a terminally differentiated organ, is incapable of undergoing mitosis and does not possess the potential to self-renew. Cardiogenesis is a developmental process that adult animals undergo (Bergmann et al., 2015). According to the cited source, it has been observed that endothelial cells exhibit a very high turnover rate, above 15% annually. In contrast, mesenchymal cells demonstrate a comparatively lower turnover rate, beyond 4% per year. Similarly, cardiomyocytes, a kind of cell found in the heart, exhibit a relatively low turnover rate, exceeding 1% annually (Bergmann et al., 2015).
There is a correlation between human chronological age and chronic heart failure, which leads to a steady deterioration in competent cardiac stem cells (CSC). These CSCs have certain characteristics such as shorter telomeres, less telomerase activity, the presence of telomere-induced foci, and an increased expression of senescence markers p21 and p16 (Cesselli et al., 2011; Chimenti et al., 2003). The disruption of telomeres in adult cardiac stem cells (CSCs) and cardiomyocytes has been suggested as a key biological mechanism behind the limited regenerative capacity of the adult mammalian heart (Aix et al., 2016; Bär et al., 2014; Cesselli et al., 2011; Chimenti et al., 2003). During embryonic development and the first days after birth, mammalian cardiomyocytes exhibit active proliferation. However, in adult individuals, cardiomyocytes that are wounded after MI lack the ability to regenerate the cardiac tissue due to a reduced capacity for proliferation (Bergmann et al., 2015; Mollova et al., 2013). The miR-15 microRNA family has been implicated in the regulation of neonatal cardiac repair via the suppression of postnatal cardiomyocyte proliferation (Porrello et al., 2013).
The role of epigenetic control on cardiomyocyte proliferation has been shown via many mechanisms, including as DNA methylation, chromatin remodeling, and histone modifications (Uygur & Lee, 2016). Mice in the adult stage that lack telomerase and possess critically shortened telomeres exhibit the manifestation of cardiomyopathy. This condition is distinguished by impaired cellular division, heightened mortality of cardiomyocytes, cellular hypertrophy, as well as ventricular dilatation, weakened heart walls, and eventual cardiac failure (Leri et al., 2003). The discovery has led to the proposition that shortened telomeres serve as one of the upstream signals that facilitate the death of cardiomyocytes.
Previous studies have shown that postnatal hypoxia, scavenging of reactive oxygen species (ROS), or inhibition of the DDR might prolong the period during which cardiomyocytes can proliferate after birth. Conversely, hyperoxemia and the presence of ROS producers have been shown to reduce this proliferative window (Puente et al., 2014). There is a potential correlation between the elevated levels of ROS after birth and the observed reduction in TL throughout the first stages of mouse development (Aix et al., 2016; Zglinicki, 2002).
Therapeutic strategies for the treatment of CVDs
Despite the notable progress made in comprehending cardiac biology, the restoration of adult tissue and functional recuperation of the adult heart after MI have yet to be effectively addressed. This underscores the need for the development of innovative treatment approaches (Martínez & Blasco, 2018). The identification of a correlation between CVDs and reduced TL presents an opportunity for the development of therapeutic interventions focused on elongating telomeres. This, in turn, might potentially restore the capacity for cell division in adult mammalian cardiomyocytes, offering promising avenues for heart regeneration after MI.
Regrettably, the therapeutic efficacy of autologous cell therapy is limited by factors such as advanced age and CVD (Dimmeler & Leri, 2008). Utilizing heterologous cells as a potential strategy to mitigate cellular damage associated with aging and disease might serve as a viable alternative. However, it is probable that this approach may elicit an immunological reaction, resulting in cellular rejection and significant hazards for the recipients undergoing treatment. The use of pretreatment including autologous cell administration has the potential to enhance the effectiveness of cell therapy in aged or medically compromised individuals by reinstating their physiological functions or regulating their cellular differentiation.
Excess neurohormonal stimulation causes telomere shortening and oxidative damage in cardiomyocytes in HF with lower ejection fraction, and this may be used to stratify HF treatment (Brandt et al., 2022).
The potential use of proliferation-based treatment has been suggested as a means to reduce oxidative stress that is reliant on mitochondria (Puente et al., 2014). Recent research has shown that hypoxemia has a therapeutic role in adult heart regeneration within a mouse model of MI. In the presence of low oxygen levels, adult cardiomyocytes undergo metabolic reprogramming, resulting in the activation of cell cycle re-entry and subsequent heart regeneration. One week after MI, the occurrence of hypoxemia triggers a notable regenerative reaction characterized by a reduction in myocardial fibrosis and an enhancement in heart function (Nakada et al., 2017).
Telomere-based therapeutic interventions
There is now a growing inclination towards the advancement of therapeutic interventions based on telomeres, with the aim of addressing age-related ailments such as telomeropathies. The chemical activator TA-65, derived from Astragalus membranaceus extracts, has been shown to improve many age-related factors (Bernardes de Jesus & Blasco, 2013; Harley et al., 1990). The mechanism of action of androgen therapy in the treatment of aplastic anaemia has been used for a considerable duration, although lacking comprehensive understanding (Shahidi & Diamond, 1961). Subsequent research demonstrated that sex hormones have the ability to initiate TERT transcription (Calado et al., 2011).
According to Puente et al. ( 2014), the implementation of a therapeutic strategy aimed at reducing mitochondrial-dependent oxidative stress might potentially serve as a feasible approach to enhance cellular proliferation. During the first stages of development, the transition from a state of low oxygen levels (hypoxia) to a state of normal oxygen levels (normoxia) results in an elevation in ROS levels. This increase in ROS has the potential to initiate DNA damage and subsequently activate the DNA damage response. The increase in levels of ROS after childbirth may also lead to reductions in TL and the occurrence of telomere losses, as shown in the first days of mouse life (Martínez & Blasco, 2018). Further investigation is required to ascertain the potential correlation between changes in neonatal cardiomyocyte proliferation induced by ROS and the maintenance of TL homeostasis. The extension of the postnatal proliferative window of cardiomyocytes has been seen by the processes of ROS scavenging or DDR inhibition. Conversely, the proliferation of cardiomyocytes is reduced by ROS generators and hyperoxia. Hoffmann et al. (2021) have identified TERT and telomerase as potential targets for addressing cardiovascular aging and its associated inflammatory signaling. This is due to the observed increase in telomerase activity resulting from the administration of statin medication and engagement in endurance exercise.
The prevailing belief is that T lymphocytes use telomerase activation as a means to delay the process of senescence. However, recent research findings indicate that some T cells, namely naïve and central memory cells, are capable of elongating telomeres without relying on telomerase activity (Weng, 2008). Instead, these cells acquire telomere vesicles from antigen-presenting cells (APCs) to achieve telomere extension. In a recent study conducted by Lanna et al. (2022), it was observed that APCs contributed telomeres through the degradation of shelterin upon interaction with T cells. Subsequently, these telomeres were cleaved by the telomere trimming factor known as telomeric-zinc-finger associated protein (TZAP) and transferred via extracellular vesicles at the immunological synapse (Lanna et al., 2022). Telomere vesicles facilitated the fusion of telomeres with the chromosomal ends of T-cells, resulting in an average extension of 3000 base pairs. Consequently, there are subsets of T lymphocytes that are unique to antigens, and their fate regarding ageing is determined by the transfer of telomere vesicles during their first contact with APCs. This transfer mechanism serves to safeguard these lymphocytes from senescence prior to the onset of clonal division, thereby providing long-lasting immune protection.
Cyanidin-3-O-glucoside (C3G), a naturally occurring compound that inhibits CD38, has shown the ability to extend human lifespan by decelerating the aging process (Zhou et al., 2022). A study [Reference Hoffmann et al., (2021)] investigated the cellular processes behind the role of Cluster of Differentiation 38 (CD38) in the process of cellular senescence, as well as explored the potential anti-aging effects of C3G. The researchers made the observation that CD38 downregulates the expression of Sirt6, leading to the induction of cell senescence. Conversely, it was shown that C3G has an anti-aging effect via the CD38-Sirt6 signaling pathway. The expression of CD38 increased in both cellular and animal models of acute aging, but the expression of Sirt6 decreased. In contrast, the application of CD38 siRNA, C3G, and UBCS039 resulted in a decrease in senescence via the reduction of ROS and SA-galactosidase levels. The administration of C3G in mice resulted in a reduction in pro-inflammatory cytokine levels, accompanied by an increase in NAD+ levels and the number of NK cells. Due to its impact on the CD38-Sirt6 signaling pathway, C3G has promising promise as a pharmaceutical intervention for anti-aging purposes.
Pharmacological methodologies targeting the restoration of the regenerative capacity of the adult mammalian heart provide intriguing prospects for the prevention and treatment of CVD. The proven advantages associated with the reactivation of telomerase make telomerase gene therapy an attractive approach for the field of heart regenerative medicine. However, more investigation is necessary before considering its use in clinical settings.
Data availability
Not applicable.
References
Aix, E., Gutiérrez-Gutiérrez, Ó., Sánchez-Ferrer, C., Aguado, T., & Flores, I. (2016). Postnatal telomere dysfunction induces cardiomyocyte cell-cycle arrest through P21 activation. Journal of Cell Biology, 213, 571–583.
Ashrafian, H., Harling, L., Darzi, A., & Athanasiou, T. (2013). Neurodegenerative disease and obesity: What is the role of weight loss and bariatric interventions? Metabolic Brain Disease, 28, 341–353.
Bär, C., De Jesus, B. B., Serrano, R., Tejera, A., Ayuso, E., Jimenez, V., Formentini, I., Bobadilla, M., Mizrahi, J., De Martino, A., et al. (2014). Telomerase expression confers cardioprotection in the adult mouse heart after acute myocardial infarction. Nature Communications, 5, 5863. https://doi.org/10.1038/ncomms6863
Bekaert, S., Van Pottelbergh, I., De Meyer, T., Zmierczak, H., Kaufman, J. M., Van Oostveldt, P., & Goemaere, S. (2005). Telomere length versus hormonal and bone mineral status in healthy elderly men. Mechanisms of Ageing and Development, 126, 1115–1122. https://doi.org/10.1016/j.mad.2005.04.007
Benetti, R., García-Cao, M., & Blasco, M. A. (2007). Telomere length regulates the epigenetic status of mammalian telomeres and subtelomeres. Nature Genetics, 39, 243–250. https://doi.org/10.1038/ng1952
Benjamin, E. J., Blaha, M. J., Chiuve, S. E., Cushman, M., Das, S. R., Deo, R., De Ferranti, S. D., Floyd, J., Fornage, M., & Gillespie, C. (2017). Heart disease and stroke statistics—2017 update: A Report from the American Heart Association. Circulation, 135, e146–e603.
Bergmann, O., Zdunek, S., Felker, A., Salehpour, M., Alkass, K., Bernard, S., Sjostrom, S. L., Szewczykowska, M., Jackowska, T., & Dos Remedios, C. (2015). Dynamics of cell generation and turnover in the human heart. Cell, 161, 1566–1575.
Bernardes de Jesus, B., & Blasco, M. A. (2013). Telomerase at the intersection of cancer and aging. Trends in Genetics, 29, 513–520. https://doi.org/10.1016/j.tig.2013.06.007
Bernardes de Jesus, B., Vera, E., Schneeberger, K., Tejera, A. M., Ayuso, E., Bosch, F., & Blasco, M. A. (2012). Telomerase gene therapy in adult and old mice delays aging and increases longevity without increasing cancer. EMBO Molecular Medicine, 4, 691–704.
Blackburn, E. H. (2000). Telomere states and cell Fates. Nature, 408, 53–56.
Blackburn, E. H. (2001). Switching and signaling at the telomere. Cell, 106, 661–673. https://doi.org/10.1016/S0092-8674(01)00492-5
Blackburn, E. H., Epel, E. S., & Lin, J. (1979). Human telomere biology: A contributory and interactive factor in aging, disease risks, and protection. Science, 2015(350), 1193–1198. https://doi.org/10.1126/science.aab3389
Blasco, M. A., Lee, H. W., Hande, M. P., Samper, E., Lansdorp, P. M., DePinho, R. A., & Greider, C. W. (1997). Telomere shortening and tumor formation by mouse cells lacking telomerase RNA. Cell, 91, 25–34. https://doi.org/10.1016/S0092-8674(01)80006-4
Bodnar, A. G., Kim, N. W., Effros, R. B., & Chiu, C. P. (1996). Mechanism of telomerase induction during T cell activation. Experimental Cell Research, 228, 58–64. https://doi.org/10.1006/excr.1996.0299
Bodnar, A. G., Ouellette, M., Frolkis, M., Holt, S. E., Chiu, C. P., Morin, G. B., Harley, C. B., Shay, J. W., Lichtsteiner, S., & Wright, W. E. (1979). Extension of life-span by introduction of telomerase into normal human cells. Science, 1998(279), 349–352. https://doi.org/10.1126/science.279.5349.349
Booth, S. A., & Charchar, F. J. (2017). Cardiac telomere length in heart development, function, and disease. Physiological Genomics, 49, 368–384. https://doi.org/10.1152/physiolgenomics.00024.2017
Booth, S. A., Wadley, G. D., Marques, F. Z., Wlodek, M. E., & Charchar, F. J. (2018). Fetal growth restriction shortens cardiac telomere length, but this is attenuated by exercise in early life. Physiological Genomics, 50, 956–963. https://doi.org/10.1152/physiolgenomics.00042.2018
Brandt, M., Dörschmann, H., Khraisat, S., Knopp, T., Ringen, J., Kalinovic, S., Garlapati, V., Siemer, S., Molitor, M., & Göbel, S. (2022). Telomere shortening in hypertensive heart disease depends on oxidative DNA damage and predicts impaired recovery of cardiac function in heart failure. Hypertension, 79, 2173–2184.
Brouilette, S. W., Moore, J. S., McMahon, A. D., Thompson, J. R., Ford, I., Shepherd, J., Packard, C. J., & Samani, N. J. (2007). Telomere length, risk of coronary heart disease, and statin treatment in the west of scotland primary prevention study: A nested case-control Study. The Lancet, 369, 107–114.
Butt, H. Z., Atturu, G., London, N. J., Sayers, R. D., & Bown, M. J. (2010). Telomere length dynamics in vascular disease: A review. European Journal of Vascular and Endovascular Surgery, 40, 17–26.
Calado, R. T., Brudno, J., Mehta, P., Kovacs, J. J., Wu, C., Zago, M. A., Chanock, S. J., Boyer, T. D., & Young, N. S. (2011). Constitutional Telomerase Mutations Are Genetic Risk Factors for Cirrhosis. Hepatology, 53, 1600–1607.
Canela, A., Vera, E., Klatt, P., & Blasco, M. A. (2007). High-throughput telomere length quantification by FISH and its application to human population studies. Proceedings of the National Academy of Sciences, 104, 5300–5305.
Cawthon, R. M., Smith, K. R., O’Brien, E., Sivatchenko, A., & Kerber, R. A. (2003). Association between telomere length in blood and mortality in people aged 60 years or older. Lancet, 361, 393–395. https://doi.org/10.1016/S0140-6736(03)12384-7
Cesselli, D., Beltrami, A. P., D’Aurizio, F., Marcon, P., Bergamin, N., Toffoletto, B., Pandolfi, M., Puppato, E., Marino, L., & Signore, S. (2011). Effects of age and heart failure on human cardiac stem cell function. American Journal of Pathology, 179, 349–366.
Chakravarti, D., LaBella, K. A., & DePinho, R. A. (2021). Telomeres: History, health, and hallmarks of aging. Cell, 184, 306–322. https://doi.org/10.1016/j.cell.2020.12.028
Chilton, W., O’Brien, B., & Charchar, F. (2017). Telomeres, aging and exercise: Guilty by association? International Journal of Molecular Sciences, 18, 2573.
Chimenti, C., Kajstura, J., Torella, D., Urbanek, K., Heleniak, H., Colussi, C., Di Meglio, F., Nadal-Ginard, B., Frustaci, A., & Leri, A. (2003). Senescence and death of primitive cells and myocytes lead to premature cardiac aging and heart failure. Circulation Research, 93, 604–613.
Codd, V., Nelson, C. P., Albrecht, E., Mangino, M., Deelen, J., Buxton, J. L., Hottenga, J. J., Fischer, K., Esko, T., & Surakka, I. (2013). Identification of seven loci affecting mean telomere length and their association with disease. Nature Genetics, 45, 422–427.
Collado, M., Blasco, M. A., & Serrano, M. (2007). Cellular senescence in cancer and aging. Cell, 130, 223–233.
D’Mello, M. J. J., Ross, S. A., Briel, M., Anand, S. S., Gerstein, H., & Paré, G. (2015). Association between shortened leukocyte telomere length and cardiometabolic outcomes: Systematic review and meta-analysis. Circulation. Cardiovascular Genetics, 8, 82–90.
Dagarag, M., Ng, H., Lubong, R., Effros, R. B., & Yang, O. O. (2003). Differential impairment of lytic and cytokine functions in senescent human immunodeficiency virus type 1-specific cytotoxic T lymphocytes. Journal of Virology, 77, 3077–3083.
de Lange, T. (2002). Protection of mammalian telomeres. Oncogene, 21, 532–540.
De Lange, T. (2005). Shelterin: The protein complex that shapes and safeguards human telomeres. Genes & Development, 19, 2100–2110. https://doi.org/10.1101/gad.1346005
de Lange, T., Shiue, L., Myers, R. M., Cox, D. R., Naylor, S. L., Killery, A. M., & Varmus, H. E. (1990). Structure and variability of human chromosome ends. Molecular and Cellular Biology, 10, 518–527. https://doi.org/10.1128/mcb.10.2.518-527.1990
De Meyer, T., Nawrot, T., Bekaert, S., De Buyzere, M. L., Rietzschel, E. R., & Andrés, V. (2018). Telomere length as cardiovascular aging biomarker: JACC review topic of the week. Journal of the American College of Cardiology, 72, 805–813.
del López-Armas, G. C., Ramos-Márquez, M. E., Navarro-Meza, M., Macías-Islas, M. Á., Saldaña-Cruz, A. M., Zepeda-Moreno, A., Siller-López, F., & Cruz-Ramos, J. A. (2023). Leukocyte telomere length predicts severe disability in relapsing-remitting multiple sclerosis and correlates with mitochondrial DNA copy number. International Journal of Molecular Sciences, 24, 916.
Dimmeler, S., & Leri, A. (2008). Aging and disease as modifiers of efficacy of cell therapy. Circulation Research, 102, 1319–1330.
Eisenberg, D. T. A. (2014). Inconsistent inheritance of telomere length (TL): Is offspring TL more strongly correlated with maternal or paternal TL? European Journal of Human Genetics, 22, 8–9.
Eisenberg, D. T. A., Salpea, K. D., Kuzawa, C. W., Hayes, M. G., & Humphries, S. E. (2011). Substantial variation in QPCR measured mean blood telomere lengths in young men from Eleven European Countries. American Journal of Human Biology, 23, 228–231. https://doi.org/10.1002/ajhb.21126
Engelhardt, M., Ozkaynak, M. F., Drullinsky, P., Sandoval, C., Tugal, O., Jayabose, S., & Moore, M. A. S. (1998). Telomerase activity and telomere length in pediatric patients with malignancies undergoing chemotherapy. Leukemia, 12, 13–24.
Entringer, S., Epel, E. S., Kumsta, R., Lin, J., Hellhammer, D. H., Blackburn, E. H., Wüst, S., & Wadhwa, P. D. (2011). Stress exposure in intrauterine life is associated with shorter telomere length in young adulthood. Proceedings of the National Academy of Sciences, 108, E513–E518.
Epel, E. S., Blackburn, E. H., Lin, J., Dhabhar, F. S., Adler, N. E., Morrow, J. D., & Cawthon, R. M. (2004). Accelerated telomere shortening in response to life stress. Proceedings of the National Academy of Sciences, 101, 17312–17315.
Epel, E. S., Merkin, S. S., Cawthon, R., Blackburn, E. H., Adler, N. E., Pletcher, M. J., & Seeman, T. E. (2009). The rate of leukocyte telomere shortening predicts mortality from cardiovascular disease in elderly Men. Aging, 1, 81–88. https://doi.org/10.18632/aging.100007
Farzaneh-Far, R., Lin, J., Epel, E. S., Harris, W. S., Blackburn, E. H., & Whooley, M. A. (2010b). Association of marine omega-3 fatty acid levels with telomeric aging in patients with coronary heart disease. JAMA, 303, 250–257.
Farzaneh-Far, R., Lin, J., Epel, E., Lapham, K., Blackburn, E., & Whooley, M. A. (2010a). Telomere length trajectory and its determinants in persons with coronary artery disease: Longitudinal Findings from the Heart and Soul Study. PLoS ONE, 5, e8612. https://doi.org/10.1371/journal.pone.0008612
Ferrón, S., Mira, H., Franco, S., Cano-Jimenez, M., Bellmunt, E., Ramírez, C., Fariñas, I., & Blasco, M. A. (2004). Telomere shortening and chromosomal instability abrogates proliferation of adult but not embryonic neural stem cells. Development, 131, 4059–4070. https://doi.org/10.1242/dev.01215
Flores, I., Canela, A., Vera, E., Tejera, A., Cotsarelis, G., & Blasco, M. A. (2008). The longest telomeres: A general signature of adult stem cell compartments. Genes & Development, 22, 654–667.
Flores, I., Cayuela, M. L., & Blasco, M. A. (1979). Molecular biology: Effects of telomerase and telomere length on epidermal stem cell behavior. Science, 2005(309), 1253–1256. https://doi.org/10.1126/science.1115025
Gardner, M., Bann, D., Wiley, L., Cooper, R., Hardy, R., Nitsch, D., Martin-Ruiz, C., Shiels, P., Sayer, A. A., Barbieri, M., et al. (2014). Gender and telomere length: Systematic review and meta-analysis. Experimental Gerontology, 51, 15–27. https://doi.org/10.1016/j.exger.2013.12.004
Griffith, J. D., Comeau, L., Rosenfield, S., Stansel, R. M., Bianchi, A., Moss, H., & De Lange, T. (1999). Mammalian telomeres end in a large duplex loop. Cell, 97, 503–514.
Guan, J. Z., Maeda, T., Sugano, M., Oyama, J., Higuchi, Y., & Makino, N. (2007). Change in the telomere length distribution with age in the Japanese population. Molecular and Cellular Biochemistry, 304, 353–360.
Haendeler, J., Hoffmann, J., Diehl, J. F., Vasa, M., Spyridopoulos, I., Zeiher, A. M., & Dimmeler, S. (2004). Antioxidants inhibit nuclear export of telomerase reverse transcriptase and delay replicative senescence of endothelial cells. Circulation Research, 94, 768–775.
Hannum, G., Guinney, J., Zhao, L., Zhang, L. I., Hughes, G., Sadda, S., Klotzle, B., Bibikova, M., Fan, J.-B., & Gao, Y. (2013). Genome-wide methylation profiles reveal quantitative views of human aging rates. Molecular Cell, 49, 359–367.
Harley, C. B., Futcher, A. B., & Greider, C. W. (1990). Telomeres shorten during ageing of human fibroblasts. Nature, 345, 458–460. https://doi.org/10.1038/345458a0
Harris, S. E., Deary, I. J., MacIntyre, A., Lamb, K. J., Radhakrishnan, K., Starr, J. M., Whalley, L. J., & Shiels, P. G. (2006). The association between telomere length, physical health, cognitive ageing, and mortality in non-demented older people. Neuroscience Letters, 406, 260–264.
Hayflick, L., & Moorhead, P. S. (1961). The serial cultivation of human diploid cell strains. Experimental Cell Research, 25, 585–621.
Hemmeryckx, B., Hohensinner, P., Swinnen, M., Heggermont, W., Wojta, J., & Lijnen, H. R. (2016). Antioxidant treatment improves cardiac dysfunction in a murine model of premature aging. Journal of Cardiovascular Pharmacology, 68, 374–382.
Herrera, E., Samper, E., Martín-Caballero, J., Flores, J. M., Lee, H.-W., & Blasco, M. A. (1999). Disease states associated with telomerase deficiency appear earlier in mice with short telomeres. EMBO Journal, 18, 2950–2960.
Hoffmann, J., Erben, Y., Zeiher, A. M., Dimmeler, S., & Spyridopoulos, I. (2009). Telomere length-heterogeneity among myeloid cells is a predictor for chronological ageing. Experimental Gerontology, 44, 363–366. https://doi.org/10.1016/j.exger.2009.02.006
Hoffmann, J., Richardson, G., Haendeler, J., Altschmied, J., Andrés, V., & Spyridopoulos, I. (2021). Telomerase as a therapeutic target in cardiovascular disease. Arteriosclerosis, Thrombosis, and Vascular Biology, 41, 1047–1061.
Hoffmann, J., & Spyridopoulos, I. (2011). Telomere length in cardiovascular disease: New challenges in measuring this marker of cardiovascular aging. Future Cardiology, 7, 693–707. https://doi.org/10.2217/fca.11.55
Hooijberg, E., Ruizendaal, J. J., Snijders, P. J. F., Kueter, E. W. M., Walboomers, J. M. M., & Spits, H. (2000). Immortalization of human CD8+ T cell clones by ectopic expression of telomerase reverse transcriptase. The Journal of Immunology, 165, 4239–4245.
Horvath, S. (2013). DNA methylation age of human tissues and cell types. Genome Biology, 14, 3156. https://doi.org/10.1186/gb-2013-14-10-r115
Iancu, E. M., Speiser, D. E., & Rufer, N. (2008). Assessing ageing of individual T lymphocytes: Mission impossible? Mechanisms of Ageing and Development, 129, 67–78.
Jakob, S., Schroeder, P., Lukosz, M., Büchner, N., Spyridopoulos, I., Altschmied, J., & Haendeler, J. (2008). Nuclear protein tyrosine phosphatase Shp-2 is one important negative regulator of nuclear export of telomerase reverse transcriptase. Journal of Biological Chemistry, 283, 33155–33161. https://doi.org/10.1074/jbc.M805138200
Jiang, R., Hauser, E. R., Kwee, L. C., Shah, S. H., Regan, J. A., Huebner, J. L., Kraus, V. B., Kraus, W. E., & Ward-Caviness, C. K. (2022). The association of accelerated epigenetic age with all-cause mortality in cardiac catheterization patients as mediated by vascular and cardiometabolic outcomes. Clinical Epigenetics, 14, 165. https://doi.org/10.1186/s13148-022-01380-x
Jin, X., Pan, B., Dang, X., Wu, H., & Xu, D. (2018). Relationship between short telomere length and stroke: A meta-analysis. Medicine 97.
Kajstura, J., Gurusamy, N., Ogórek, B., Goichberg, P., Clavo-Rondon, C., Hosoda, T., D’Amario, D., Bardelli, S., Beltrami, A. P., & Cesselli, D. (2010). Myocyte turnover in the aging human heart. Circulation Research, 107, 1374–1386.
Kaur, P., Wu, D., Lin, J., Countryman, P., Bradford, K. C., Erie, D. A., Riehn, R., Opresko, P. L., & Wang, H. (2016). Enhanced electrostatic force microscopy reveals higher-order DNA looping mediated by the telomeric protein TRF2. Science and Reports, 6, 20513.
Khincha, P. P., Bertuch, A. A., Agarwal, S., Townsley, D. M., Young, N. S., Keel, S., Shimamura, A., Boulad, F., Simoneau, T., & Justino, H. (2017). Pulmonary arteriovenous malformations: An uncharacterised phenotype of dyskeratosis congenita and related telomere biology disorders. European Respiratory Journal 49.
Kovalenko, O. A., Caron, M. J., Ulema, P., Medrano, C., Thomas, A. P., Kimura, M., Bonini, M. G., Herbig, U., & Santos, J. H. (2010). A mutant telomerase defective in nuclear-cytoplasmic shuttling fails to immortalize cells and is associated with mitochondrial dysfunction. Aging Cell, 9, 203–219. https://doi.org/10.1111/j.1474-9726.2010.00551.x
Kuhlow, D., Florian, S., von Figura, G., Weimer, S., Schulz, N., Petzke, K. J., Zarse, K., Pfeiffer, A. F. H., Rudolph, K. L., & Ristow, M. (2010). Telomerase deficiency impairs glucose metabolism and insulin secretion. Aging (albany NY), 2, 650.
Lanna, A., Vaz, B., D’Ambra, C., Valvo, S., Vuotto, C., Chiurchiù, V., Devine, O., Sanchez, M., Borsellino, G., Akbar, A. N., et al. (2022). An intercellular transfer of telomeres rescues T cells from senescence and promotes long-term immunological memory. Nature Cell Biology, 24, 1461–1474. https://doi.org/10.1038/s41556-022-00991-z
Lansdorp, P. M. (1998). Self-renewal of stem cells. Humana Press.
Lee, H.-W., Blasco, M. A., Gottlieb, G. J., Horner, J. W., Greider, C. W., & DePinho, R. A. (1998). Essential role of mouse telomerase in highly proliferative organs. Nature, 392, 569–574.
Leri, A., Franco, S., Zacheo, A., Barlucchi, L., Chimenti, S., Limana, F., Nadal-Ginard, B., Kajstura, J., Anversa, P., & Blasco, M. A. (2003). Ablation of telomerase and telomere loss leads to cardiac dilatation and heart failure associated with P53 upregulation. EMBO Journal, 22, 131–139.
Lindsey, J., McGill, N. I., Lindsey, L. A., Green, D. K., & Cooke, H. J. (1991). In vivo loss of telomeric repeats with age in humans. Mutation Research DNAging, 256, 45–48. https://doi.org/10.1016/0921-8734(91)90032-7
Liu, D., O’Connor, M. S., Qin, J., & Songyang, Z. (2004). Telosome, a mammalian telomere-associated complex formed by multiple telomeric proteins. Journal of Biological Chemistry, 279, 51338–51342.
Liu, L., Bailey, S. M., Okuka, M., Muñoz, P., Li, C., Zhou, L., Wu, C., Czerwiec, E., Sandler, L., & Seyfang, A. (2007). telomere lengthening early in development. Nature Cell Biology, 9, 1436–1441.
López-Otín, C., Blasco, M. A., Partridge, L., Serrano, M., & Kroemer, G. (2013). The hallmarks of aging. Cell, 153, 1194–1217.
Ludlow, A. T., Witkowski, S., Marshall, M. R., Wang, J., Lima, L. C. J., Guth, L. M., Spangenburg, E. E., & Roth, S. M. (2012). Chronic exercise modifies age-related telomere dynamics in a tissue-specific fashion. Journals of Gerontology-Series A Biological Sciences and Medical Sciences, 67A, 911–926. https://doi.org/10.1093/gerona/gls002
Lynch, S. M., Peek, M. K., Mitra, N., Ravichandran, K., Branas, C., Spangler, E., Zhou, W., Paskett, E. D., Gehlert, S., Degraffinreid, C., et al. (2016). Race, ethnicity, psychosocial factors, and telomere length in a multicenter setting. PLoS ONE, 11, e0146723. https://doi.org/10.1371/journal.pone.0146723
Marion, R. M., Strati, K., Li, H., Tejera, A., Schoeftner, S., Ortega, S., Serrano, M., & Blasco, M. A. (2009). Telomeres acquire embryonic stem cell characteristics in induced pluripotent stem cells. Cell Stem Cell, 4, 141–154. https://doi.org/10.1016/j.stem.2008.12.010
Marques, F. Z., Booth, S. A., Prestes, P. R., Curl, C. L., Delbridge, L. M. D., Lewandowski, P., Harrap, S. B., & Charchar, F. J. (2016). Telomere dynamics during aging in polygenic left ventricular hypertrophy. Physiological Genomics, 48, 42–49.
Martínez, P., & Blasco, M. A. (2011). Telomeric and extra-telomeric roles for telomerase and the telomere-binding proteins. Nature Reviews Cancer, 11, 161–176. https://doi.org/10.1038/nrc3025
Martínez, P., & Blasco, M. A. (2018). Heart-breaking telomeres. Circulation Research, 123, 787–802.
Mather, K. A., Jorm, A. F., Milburn, P. J., Tan, X., Easteal, S., & Christensen, H. (2010). No associations between telomere length and age-sensitive indicators of physical function in mid and later life. Journals of Gerontology-Series A Biological Sciences and Medical Sciences, 65A, 792–799. https://doi.org/10.1093/gerona/glq050
Matthews, C., Gorenne, I., Scott, S., Figg, N., Kirkpatrick, P., Ritchie, A., Goddard, M., & Bennett, M. (2006). Vascular smooth muscle cells undergo telomere-based senescence in human atherosclerosis: Effects of telomerase and oxidative stress. Circulation Research, 99, 156–164.
McCully, K. S. (2018). Chemical pathology of homocysteine VI. Aging, cellular senescence, and mitochondrial dysfunction. Annals of Clinical Laboratory Science, 48, 677–687.
Mollova, M., Bersell, K., Walsh, S., Savla, J., Das, L. T., Park, S.-Y., Silberstein, L. E., Dos Remedios, C. G., Graham, D., & Colan, S. (2013). Cardiomyocyte proliferation contributes to heart growth in young humans. Proceedings of the National Academy of Sciences, 110, 1446–1451.
Mwasongwe, S., Gao, Y., Griswold, M., Wilson, J. G., Aviv, A., Reiner, A. P., & Raffield, L. M. (2017). Leukocyte telomere length and cardiovascular disease in African Americans: The Jackson Heart Study. Atherosclerosis, 266, 41–47. https://doi.org/10.1016/j.atherosclerosis.2017.09.016
Nakada, Y., Canseco, D. C., Thet, S., Abdisalaam, S., Asaithamby, A., Santos, C. X., Shah, A. M., Zhang, H., Faber, J. E., & Kinter, M. T. (2017). Hypoxia induces heart regeneration in adult mice. Nature, 541, 222–227.
Narducci, M. L., Grasselli, A., Biasucci, L. M., Farsetti, A., Mulè, A., Liuzzo, G., La Torre, G., Niccoli, G., Mongiardo, R., & Pontecorvi, A. (2007). High telomerase activity in neutrophils from unstable coronary plaques. Journal of the American College of Cardiology, 50, 2369–2374.
Njajou, O. T., Hsueh, W.-C., Blackburn, E. H., Newman, A. B., Wu, S.-H., Li, R., Simonsick, E. M., Harris, T. M., Cummings, S. R., & Cawthon, R. M. (2009). Association between telomere length, specific causes of death, and years of healthy life in health, aging, and body composition, a population-based cohort study. Journals of Gerontology Series a: Biomedical Sciences and Medical Sciences, 64, 860–864.
O’Donovan, A., Epel, E., Lin, J., Wolkowitz, O., Cohen, B., Maguen, S., Metzler, T., Lenoci, M., Blackburn, E., & Neylan, T. C. (2011b). Childhood trauma associated with short leukocyte telomere length in posttraumatic stress disorder. Biological Psychiatry, 70, 465–471. https://doi.org/10.1016/j.biopsych.2011.01.035
O’Donovan, A., Pantell, M. S., Puterman, E., Dhabhar, F. S., Blackburn, E. H., Yaffe, K., Cawthon, R. M., Opresko, P. L., Hsueh, W. C., Satterfield, S., et al. (2011a). Cumulative inflammatory load is associated with short leukocyte telomere length in the health, aging and body composition study. PLoS ONE, 6, e19687. https://doi.org/10.1371/journal.pone.0019687
Ogami, M., Ikura, Y., Ohsawa, M., Matsuo, T., Kayo, S., Yoshimi, N., Hai, E., Shirai, N., Ehara, S., & Komatsu, R. (2004). Telomere shortening in human coronary artery diseases. Arteriosclerosis, Thrombosis, and Vascular Biology, 24, 546–550.
Oikawa, S., Tada-Oikawa, S., & Kawanishi, S. (2001). Site-specific DNA damage at the GGG sequence by UVA involves acceleration of telomere shortening. Biochemistry, 40, 4763–4768. https://doi.org/10.1021/bi002721g
Okuda, K., Khan, M. Y., Skurnick, J., Kimura, M., Aviv, H., & Aviv, A. (2000). Telomere attrition of the human abdominal aorta: Relationships with age and atherosclerosis. Atherosclerosis, 152, 391–398.
Olovnikov, A. M. (1973). A theory of marginotomy: The incomplete copying of template margin in enzymic synthesis of polynucleotides and biological significance of the phenomenon. Journal of Theoretical Biology, 41, 181–190.
Park, J. I. I., Venteicher, A. S., Hong, J. Y., Choi, J., Jun, S., Shkreli, M., Chang, W., Meng, Z., Cheung, P., Ji, H., et al. (2009). telomerase modulates wnt signalling by association with target gene chromatin. Nature, 460, 66–72. https://doi.org/10.1038/nature08137
Passos, J. F., Saretzki, G., & Von Zglinicki, T. (2007). DNA damage in telomeres and mitochondria during cellular senescence: Is there a connection? Nucleic Acids Research, 35, 7505–7513. https://doi.org/10.1093/nar/gkm893
Perna, L., Zhang, Y., Mons, U., Holleczek, B., Saum, K.-U., & Brenner, H. (2016). Epigenetic age acceleration predicts cancer, cardiovascular, and all-cause mortality in a German case cohort. Clinical Epigenetics, 8, 1–7. https://doi.org/10.1186/s13148-016-0228-z
Peters, M. J., Joehanes, R., Pilling, L. C., Schurmann, C., Conneely, K. N., Powell, J., Reinmaa, E., Sutphin, G. L., Zhernakova, A., Schramm, K., et al. (2015). The transcriptional landscape of age in human peripheral blood. Nature Communications, 6, 8570. https://doi.org/10.1038/ncomms9570
Petersen, S., Saretzki, G., & von Zglinicki, T. (1998). Preferential accumulation of single-stranded regions in telomeres of human fibroblasts. Experimental Cell Research, 239, 152–160.
Porrello, E. R., Mahmoud, A. I., Simpson, E., Johnson, B. A., Grinsfelder, D., Canseco, D., Mammen, P. P., Rothermel, B. A., Olson, E. N., & Sadek, H. A. (2013). Regulation of neonatal and adult mammalian heart regeneration by the MiR-15 family. Proceedings of the National Academy of Sciences, 110, 187–192.
Price, L. H., Kao, H.-T., Burgers, D. E., Carpenter, L. L., & Tyrka, A. R. (2013). Telomeres and early-life stress: An overview. Biological Psychiatry, 73, 15–23.
Proctor, C. J., & Kirkwood, T. B. L. (2002). Modelling telomere shortening and the role of oxidative stress. Mechanisms of Ageing and Development, 123, 351–363. https://doi.org/10.1016/S0047-6374(01)00380-3
Puente, B. N., Kimura, W., Muralidhar, S. A., Moon, J., Amatruda, J. F., Phelps, K. L., Grinsfelder, D., Rothermel, B. A., Chen, R., & Garcia, J. A. (2014). The oxygen-rich postnatal environment induces cardiomyocyte cell-cycle arrest through DNA damage response. Cell, 157, 565–579.
Quach, A., Levine, M. E., Tanaka, T., Lu, A. T., Chen, B. H., Ferrucci, L., & Horvath, S. (2018). Epigenetic clock analysis of diet, exercise, education, and lifestyle factors. Agro Food Industry Hi-Tech, 29, 20–21.
Révész, D., Milaneschi, Y., Verhoeven, J. E., Lin, J., & Penninx, B. W. J. H. (2015). Longitudinal associations between metabolic syndrome components and telomere shortening. Journal of Clinical Endocrinology and Metabolism, 100, 3050–3059. https://doi.org/10.1210/JC.2015-1995
Robin, J. D., Ludlow, A. T., Batten, K., Magdinier, F., Stadler, G., Wagner, K. R., Shay, J. W., & Wright, W. E. (2014). Telomere position effect: Regulation of gene expression with progressive telomere shortening over long distances. Genes & Development, 28, 2464–2476.
Rufer, N., Brümmendorf, T. H., Kolvraa, S., Bischoff, C., Christensen, K., Wadsworth, L., Schulzer, M., & Lansdorp, P. M. (1999). Telomere fluorescence measurements in granulocytes and T lymphocyte subsets point to a high turnover of hematopoietic stem cells and memory T cells in early childhood. Journal of Experimental Medicine, 190, 157–168.
Rufer, N., Migliaccio, M., Antonchuk, J., Humphries, R. K., Roosnek, E., & Lansdorp, P. M. (2001). Transfer of the human telomerase reverse transcriptase (TERT) gene into T lymphocytes results in extension of replicative potential. Blood, 98, 597–603. https://doi.org/10.1182/blood.V98.3.597
Sahin, E., Colla, S., Liesa, M., Moslehi, J., Müller, F. L., Guo, M., Cooper, M., Kotton, D., Fabian, A. J., & Walkey, C. (2011). Telomere dysfunction induces metabolic and mitochondrial compromise. Nature, 470, 359–365.
Samani, N. J., Boultby, R., Butler, R., Thompson, J. R., & Goodall, A. H. (2001). Telomere shortening in atherosclerosis. Lancet, 358, 472–473. https://doi.org/10.1016/S0140-6736(01)05633-1
Sandell, L. L., & Zakian, V. A. (1993). Loss of a yeast telomere: Arrest, recovery, and chromosome loss. Cell, 75, 729–739.
Sanders, J. L., Cauley, J. A., Boudreau, R. M., Zmuda, J. M., Strotmeyer, E. S., Opresko, P. L., Hsueh, W. C., Cawthon, R. M., Li, R., Harris, T. B., et al. (2009). Leukocyte telomere length is not associated with BMD, osteoporosis, or fracture in older adults: Results from the health, aging and body composition study. Journal of Bone and Mineral Research, 24, 1531–1536. https://doi.org/10.1359/jbmr.090318
Sanders, J. L., & Newman, A. B. (2013). Telomere length in epidemiology: A biomarker of aging, age-related disease, both, or neither? Epidemiologic Reviews, 35, 112–131. https://doi.org/10.1093/epirev/mxs008
Saretzki, G., Murphy, M. P., & Von Zglinicki, T. (2003). MitoQ counteracts telomere shortening and elongates lifespan of fibroblasts under mild oxidative stress. Aging Cell, 2, 141–143.
Sarin, K. Y., Cheung, P., Gilison, D., Lee, E., Tennen, R. I., Wang, E., Artandi, M. K., Oro, A. E., & Artandi, S. E. (2005). Conditional telomerase induction causes proliferation of hair follicle stem cells. Nature, 436, 1048–1052. https://doi.org/10.1038/nature03836
Schaetzlein, S., Lucas-Hahn, A., Lemme, E., Kues, W. A., Dorsch, M., Manns, M. P., Niemann, H., & Rudolph, K. L. (2004). Telomere length is reset during early mammalian embryogenesis. Proceedings of the National Academy of Sciences, 101, 8034–8038.
Scheller Madrid, A., Rode, L., Nordestgaard, B. G., & Bojesen, S. E. (2016). Short Telomere Length and Ischemic Heart Disease: Observational and Genetic Studies in 290 022 Individuals. Clinical Chemistry, 62, 1140–1149.
Shahidi, N. T., & Diamond, L. K. (1961). Testosterone-induced remission in aplastic anemia of both acquired and congenital types: Further observations in 24 cases. New England Journal of Medicine, 264, 953–967.
Shammas, M. A. (2011). Telomeres, lifestyle, cancer, and aging. Current Opinion in Clinical Nutrition and Metabolic Care, 14, 28.
Sharifi-Sanjani, M., Oyster, N. M., Tichy, E. D., Bedi, K. C., Jr., Harel, O., Margulies, K. B., & Mourkioti, F. (2017). Cardiomyocyte-specific telomere shortening is a distinct signature of heart failure in humans. Journal of the American Heart Association, 6, e005086.
Smith, J. A., Raisky, J., Ratliff, S. M., Liu, J., Kardia, S. L. R., Turner, S. T., Mosley, T. H., & Zhao, W. (2019). Intrinsic and extrinsic epigenetic age acceleration are associated with hypertensive target organ damage in older african americans. BMC Medical Genomics, 12, 141. https://doi.org/10.1186/s12920-019-0585-5
Stadler, G., Rahimov, F., King, O. D., Chen, J. C. J., Robin, J. D., Wagner, K. R., Shay, J. W., Emerson, C. P., Jr., & Wright, W. E. (2013). Telomere position effect regulates DUX4 in human facioscapulohumeral muscular dystrophy. Nature Structural & Molecular Biology, 20, 671–678.
Starkweather, A. R., Alhaeeri, A. A., Montpetit, A., Brumelle, J., Filler, K., Montpetit, M., Mohanraj, L., Lyon, D. E., & Jackson-Cook, C. K. (2014). An integrative review of factors associated with telomere length and implications for biobehavioral research. Nursing Research, 63, 36–50. https://doi.org/10.1097/NNR.0000000000000009
Stefler, D., Malyutina, S., Maximov, V., Orlov, P., Ivanoschuk, D., Nikitin, Y., Gafarov, V., Ryabikov, A., Voevoda, M., Bobak, M., et al. (2018). Leukocyte telomere length and risk of coronary heart disease and stroke mortality: Prospective evidence from a Russian Cohort. Science and Reports, 8, 16627. https://doi.org/10.1038/s41598-018-35122-y
Strandberg, T. E., Saijonmaa, O., Tilvis, R. S., Pitkälä, K. H., Strandberg, A. Y., Miettinen, T. A., & Fyhrquist, F. (2011b). Association of telomere length in older men with mortality and midlife body mass index and smoking. Journals of Gerontology - Series A Biological Sciences and Medical Sciences, 66A, 815–820. https://doi.org/10.1093/gerona/glr064
Strandberg, T. E., Saijonmaa, O., Tilvis, R. S., Pitkälä, K. H., Strandberg, A. Y., Salomaa, V., Miettinen, T. A., & Fyhrquist, F. (2011a). Telomere length in old age and cholesterol across the life course. Journal of the American Geriatrics Society, 59, 1979–1981. https://doi.org/10.1111/j.1532-5415.2011.03610_13.x
Svačina, Š. (2020). Obesity and cardiovascular disease. Vnitrni Lekarstvi, 66, 89–91. https://doi.org/10.1161/01.atv.0000216787.85457.f3
Tang, N. L. S., Woo, J., Suen, E. W. C., Liao, C. D., Leung, J. C. S., & Leung, P. C. (2010). The effect of telomere length, a marker of biological aging, on bone mineral density in elderly population. Osteoporosis International, 21, 89–97.
Terai, M., Izumiyama-Shimomura, N., Aida, J., Ishikawa, N., Sawabe, M., Arai, T., Fujiwara, M., Ishii, A., Nakamura, K., & Takubo, K. (2013). Association of telomere shortening in myocardium with heart weight gain and cause of death. Science and Reports, 3, 2401.
Uygur, A., & Lee, R. T. (2016). Mechanisms of cardiac regeneration. Developmental Cell, 36, 362–374.
Valdes, A. M., Andrew, T., Gardner, J. P., Kimura, M., Oelsner, E., Cherkas, L. F., Aviv, A., & Spector, T. D. (2005). Obesity, cigarette smoking, and telomere length in women. Lancet, 366, 662–664. https://doi.org/10.1016/S0140-6736(05)66630-5
Valdes, A. M., Richards, J. B., Gardner, J. P., Swaminathan, R., Kimura, M., Xiaobin, L., Aviv, A., & Spector, T. D. (2007). Telomere length in leukocytes correlates with bone mineral density and is shorter in women with osteoporosis. Osteoporosis International, 18, 1203–1210. https://doi.org/10.1007/s00198-007-0357-5
Valenzuela, H. F., & Effros, R. B. (2002). Divergent telomerase and CD28 expression patterns in human CD4 and CD8 T cells following repeated encounters with the same antigenic stimulus. Clinical Immunology, 105, 117–125. https://doi.org/10.1006/clim.2002.5271
van der Harst, P., van der Steege, G., de Boer, R. A., Voors, A. A., Hall, A. S., Mulder, M. J., van Gilst, W. H., & van Veldhuisen, D. J. (2007). Telomere length of circulating leukocytes is decreased in patients with chronic heart failure. Journal of the American College of Cardiology, 49, 1459–1464. https://doi.org/10.1016/j.jacc.2007.01.027
Vera, E., Canela, A., Fraga, M. F., Esteller, M., & Blasco, M. A. (2008). Epigenetic regulation of telomeres in human cancer. Oncogene, 27, 6817–6833. https://doi.org/10.1038/onc.2008.289
Verde, Z., Reinoso-Barbero, L., Chicharro, L., Garatachea, N., Resano, P., Sánchez-Hernández, I., Rodríguez González-Moro, J. M., Bandrés, F., Santiago, C., & Gómez-Gallego, F. (2015). Effects of cigarette smoking and nicotine metabolite ratio on leukocyte telomere length. Environmental Research, 140, 488–494. https://doi.org/10.1016/j.envres.2015.05.008
Verhulst, S., Dalgård, C., Labat, C., Kark, J. D., Kimura, M., Christensen, K., Toupance, S., Aviv, A., Kyvik, K. O., & Benetos, A. (2016). A short leucocyte telomere length is associated with development of insulin resistance. Diabetologia, 59, 1258–1265.
Von Zglinicki, T. (2002). Oxidative stress shortens telomeres. Trends in Biochemical Sciences, 27, 339–344.
Von Zglinicki, T., Pilger, R., & Sitte, N. (2000). Accumulation of single-strand breaks is the major cause of telomere shortening in human fibroblasts. Free Radical Biology & Medicine, 28, 64–74. https://doi.org/10.1016/S0891-5849(99)00207-5
Waring, C. D., Vicinanza, C., Papalamprou, A., Smith, A. J., Purushothaman, S., Goldspink, D. F., Nadal-Ginard, B., Torella, D., & Ellison, G. M. (2014). The adult heart responds to increased workload with physiologic hypertrophy, cardiac stem cell activation, and new myocyte formation. European Heart Journal, 35, 2722–2731.
Weischer, M., Bojesen, S. E., & Nordestgaard, B. G. (2014). Telomere shortening unrelated to smoking, body weight, physical activity, and alcohol intake: 4,576 general population individuals with repeat measurements 10 years apart. PLoS Genetics, 10, e1004191.
Weng, N. (2008). Telomere and adaptive immunity. Mechanisms of Ageing and Development, 129, 60–66. https://doi.org/10.1016/j.mad.2007.11.005
Weng, N.-P., Levine, B. L., June, C. H., & Hodes, R. J. (1996). Regulated expression of telomerase activity in human T lymphocyte development and activation. Journal of Experimental Medicine, 183, 2471–2479.
Werner, C., Fürster, T., Widmann, T., Pöss, J., Roggia, C., Hanhoun, M., Scharhag, J., Büchner, N., Meyer, T., & Kindermann, W. (2009). Physical exercise prevents cellular senescence in circulating leukocytes and in the vessel wall. Circulation, 120, 2438–2447.
Wong, J. Y. Y., De Vivo, I., Lin, X., Fang, S. C., & Christiani, D. C. (2014). The relationship between inflammatory biomarkers and telomere length in an occupational prospective cohort study. PLoS ONE, 9, e87348. https://doi.org/10.1371/journal.pone.0087348
Xiong, L., Yang, G., Guo, T., Zeng, Z., Liao, T., Li, Y., Li, Y., Chen, F., Yang, S., Kang, L., et al. (2023). 17-Year follow-up of association between telomere length and all-cause mortality, cardiovascular mortality in individuals with metabolic syndrome: Results from the NHANES Database Prospective Cohort Study. Diabetology and Metabolic Syndrome, 15, 247. https://doi.org/10.1186/s13098-023-01206-7
Xu, C., Wang, Z., Su, X., Da, M., Yang, Z., Duan, W., & Mo, X. (2020). Association between leucocyte telomere length and cardiovascular disease in a large general population in the United States. Science and Reports, 10, 80. https://doi.org/10.1038/s41598-019-57050-1
Zaragoza, C., Gomez-Guerrero, C., Martin-Ventura, J. L., Blanco-Colio, L., Lavin, B., Mallavia, B., Tarin, C., Mas, S., Ortiz, A., & Egido, J. (2011). Animal models of cardiovascular diseases. Biomedicine Research International, 2011, 1–13.
Zglinicki, Tv., & Martin-Ruiz, C. M. (2005). Telomeres as biomarkers for ageing and age-related diseases. Current Molecular Medicine, 5, 197–203.
Zhang, K., Ma, Y., Luo, Y., Song, Y., Xiong, G., Ma, Y., Sun, X., & Kan, C. (2023). Metabolic diseases and healthy aging: Identifying environmental and behavioral risk factors and promoting public health. Frontiers in Public Health. https://doi.org/10.3389/fpubh.2023.1253506
Zhou, H., Liu, S., Zhang, N., Fang, K., Zong, J., An, Y., & Chang, X. (2022). Downregulation of Sirt6 by CD38 Promotes Cell Senescence and Aging. Aging (albany NY), 14, 9730.
Zurek, M., Altschmied, J., Kohlgrüber, S., Ale-Agha, N., & Haendeler, J. (2016). Role of telomerase in the cardiovascular system. Genes (basel), 7, 29. https://doi.org/10.3390/genes7060029
Funding
Not applicable.
Author information
Authors and Affiliations
Biochemistry Department, Faculty of Science, Beni-Suef University, Beni-Suef, 62521, Egypt
Mohammed Abdel-Gabbar & Mohamed G. M. Kordy
Nanophotonics and Applications (NPA) Lab, Physics Department, Faculty of Science, Beni-Suef University, Beni-Suef, 62514, Egypt
Mohamed G. M. Kordy
Contributions
The authors affirm their contribution to the work in the following manner: The study's concept and design were conducted by MA-G, while MA-G was responsible for data collecting. The analysis and interpretation of findings were carried out by both MA-G and MGMK. The first draft of the paper was prepared by MA-G and MGMK. The findings were evaluated by all authors and the final version of the paper was approved.
Corresponding author
Correspondence to Mohamed G. M. Kordy.
Ethics declarations
Conflict of interest
The authors declare that they do not own any apparent conflicting financial interests or personal ties that would have been seen as exerting an impact on the research presented in this manuscript.
Ethical approval
This work does not include any research using animal or human subjects conducted by any of the authors.
Rights and permissions
Springer Nature or its licensor (e.g. a society or other partner) holds exclusive rights to this article under a publishing agreement with the author(s) or other rightsholder(s); author self-archiving of the accepted manuscript version of this article is solely governed by the terms of such publishing agreement and applicable law.
About this article
Cite this article
Abdel-Gabbar, M., Kordy, M.G.M. Telomere-based treatment strategy of cardiovascular diseases: imagination comes to reality. GENOME INSTAB. DIS. (2024). https://doi.org/10.1007/s42764-024-00123-x
Received15 November 2023
Revised09 January 2024
Accepted22 January 2024
Published16 February 2024
DOIhttps://doi.org/10.1007/s42764-024-00123-x
Share this article
Anyone you share the following link with will be able to read this content:
Get shareable link
用户登录
还没有账号?
立即注册